Plutonium is an important and highly complex element in which spontaneous self-irradiation drives lattice defects that damage the bulk solid over time. This damage includes lattice interstitials, vacancies, and defect accumulation, e.g., helium bubbles and other daughter product impurities. X-ray diffraction and dilatometry studies have revealed that the room temperature self-irradiation of δ-phase Pu-Ga alloys results in swelling of the lattice that saturates after 0.1–0.2 displacements per atom (dpa) of accumulated lattice damage. For a more complete picture of the less-understood aging processes of defect accumulation and damage evolution in δ-phase Pu-Ga alloys, we must consider additional factors such as dose, dose rate, thermal history, and alloy composition.
This article describes a time-of-flight (TOF) neutron diffraction investigation of the self-irradiation effects in δ-phase 239Pu-Ga alloys, with various histories of annealing, ambient temperature storage times, and cryogenic temperature exposure. The observed self-irradiation at ambient conditions is explained in terms of damage to long-range order and associated lattice strain.
Overview of plutonium
In May 1941, Joseph W. Kennedy, Glenn T. Seaborg, Emilio Segre, and Arthur C. Wahl reported the fission of element-94239 with slow neutrons in an article submitted to Physical Review, and published several years later in 1946. The new element, subsequently named plutonium, discovered at Berkeley’s Radiation Laboratory in 1940 showed an alpha-activity of about 60,000 alpha emissions per minute which corresponded to a half-life of about 30,000 years.
Plutonium proved to be the most unusual element of the periodic table, highly unstable with no less than six allotropes from room temperature to its melting point of 640°C. Many phases of elemental plutonium metal are sensitive to temperature, pressure, and time, which strongly affects the thermodynamic and mechanical properties and crystallographic structure in ways that are not yet completely described or even understood.
Of particular interest for technological applications is the face-centered cubic (fcc) δ-phase which exhibits unusual properties such as negative thermal expansion, large low-temperature electronic specific heat, high electrical resistivity, and other unusual behaviors. This high-temperature δ-phase, stable from 320–450°C, can be achieved at room temperature and below by the addition of a small proportion of certain trivalent elements (i.e., Ga, Al, Ce, Am, or Sc). The alloying process affects the lattice parameters and other physical properties correlated to the part of the electronic structure which is determined by the 5f electron state. It is further convoluted by the dual nature of 5f electron localization and bonding. The resulting alloys are metastable and more easily machined than the other phases, which is a very useful and important property for these materials.
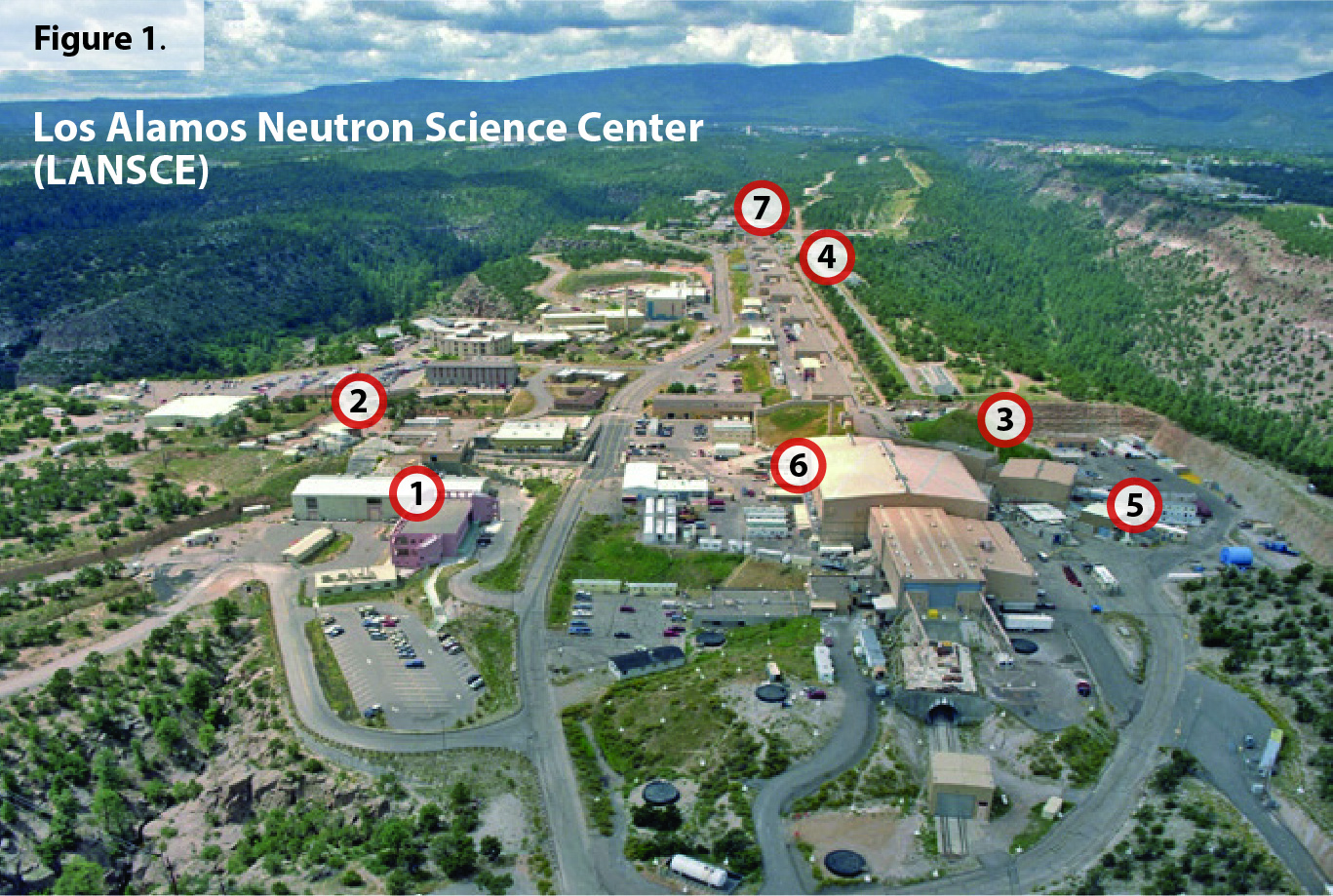
1 Lujan Neutron Scattering Center: Neutrons used in engineering, chemistry, nanotechnology, and the materials, biological, medical, and geological sciences.
2 Weapons Neutron Research (WNR): Nuclear data crucial to the Stockpile Stewardship Program in addition to other basic, applied, and defense-related research.
3 Proton Radiography: 800-MeV protons for imaging dyamic experiments in support of national and international weapons science and thr Stockpile Stewardship Program.
4 Isotope Production Facility: Radioactive isotopes for medicine and research (strontium-82 is produced to aid 20,000 heart patients each month).
5 Ultracold Neutrons: Intense, ultracold needed for experiments on the fundamental laws of physics and aid in the quest for new particles.
6 Materials Test Station (MTS): Materials and fuels testing for use in advanced fast reactors.
7 Linear Accelerator: Facilities support.
Most fcc alloys exposed to neutron irradiation and fission gases undergo changes in the microstructure (e.g., lattice distortions, steady-state void swelling rates of 0.8–1.5% dpa/yr, or changes to physical and mechanical properties). The fcc δ-phase Pu-Ga alloys experience self-irradiation with a defect production rate of 0.1 dpa/yr and helium accumulation. Consequently, they exhibit helium bubble formation and lattice/long-range order swelling to a saturated value of 0.1% at 0.2 dpa, but with minimal changes to the microstructure.
The large quantities of lattice damage in δ-phase Pu alloys induced by self-irradiation over thousands of hours at cryogenic temperatures are usually annealed when returned to ambient conditions. However, results from various ambient temperature investigations emphasize the influence of structural changes induced by self-irradiation, along with the stress and strain field changes in δ-phase Pu alloys.
These structural changes induced by radiation damage, detectable by means of X-ray or neutron diffraction, provide valuable information about the impact of aging or self-irradiation effects on the long-range order of the material (such as lattice strain and crystallinity). They also act as a measure of disorder and second phase inclusions. Compared to X-rays, neutrons have the added advantage of being highly penetrating and non-destructive, which makes them a more suitable probe for bulk measurements, and ideal for investigations that require massive experimental setups, such as those used in temperature and pressure studies.
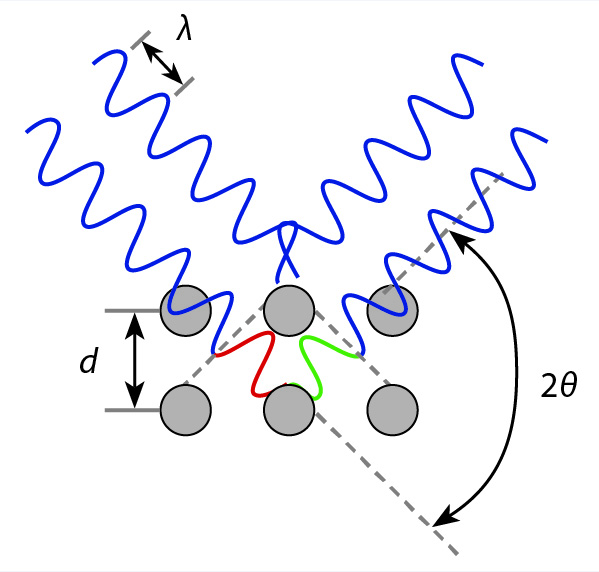
A form of elastic scattering, diffraction is a powerful nondestructive technique used to study crystalline materials to determine the static structure (from lattice parameters, phase composition, grain size, stress and strain, preferred orientation, crystallinity/amorphicity to thermal expansion, and phase transitions). X-rays and neutrons are diffracted by crystalline materials according to Bragg’s law, the result being a pattern that shows absolute intensity as a function of the diffraction angles (2θ) or the d-spacing. From its peaks (shape, intensity, position), the diffraction pattern provides valuable information about the crystal structure, the unit cell, d spacing, the crystallite size, stress, texture, and defects. It is specific to the material being measured.
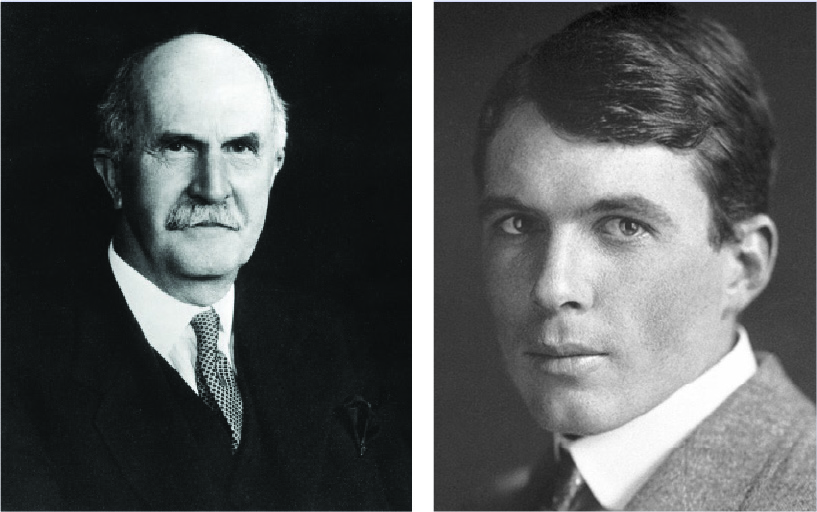
Neutron scattering
Neutron scattering is a condensed matter technique that provides information on the structure, dynamics, and magnetic properties of materials. It encompasses a large array of methods, such as diffraction, pair distribution function analysis, small-angle scattering, reflectometry, and inelastic scattering.
X-ray diffraction was an important part of the first efforts of characterizing plutonium’s structures, establishing the space group and lattice parameters of its allotropes, and understanding its behavior under non-ambient conditions such as variable temperatures and pressures. In the early 1940s, X-ray diffraction was the only analytical tool available for these type of investigations. The pioneering work of W. H. Zachariasen, considered the father of plutonium crystal chemistry, is well known and broadly used today, providing identification for most of the plutonium phases, and the first one to identify the six allotropes of Pu (see ARQ First Quarter 2020, “Reflections of Plutonium— In Search of Solutions to a Difficult Problem in Crystallography”, pp 56–60).
Neutron diffraction, performed at neutron reactors (with constant wavelength) and at spallation neutron sources (with TOF method), came into play later. The first neutron diffraction experiments were carried out in 1936 (versus 1912 for X-ray diffraction) using low intensity neutron sources. During the Manhattan Project, the construction of nuclear reactors enabled neutron beams of higher intensities which were more suitable for neutron scattering experiments. The use of neutrons as a diffraction probe was established at the University of Chicago Metallurgical Laboratory, using the Chicago Pile 3 heavy water reactor, and at Oak Ridge National Laboratory (ORNL), using the X 10 graphite reactor. Later, neutron scattering research increased significantly with the development of spallation neutron sources. The Manuel Lujan Jr. Neutron Scattering Center (Lujan Center), part of the Los Alamos Neutron Science Center (LANSCE) at Los Alamos National Laboratory (LANL) is the only neutron facility in the US that accepts highly radioactive samples, such as plutonium, and can accommodate both unclassified and classified research. As a probe, neutrons have several advantages over X-rays:
- Highly penetrating. This makes them a bulk probe and suitable for massive experimental setup (e.g., high pressure and non-ambient temperature experiments).
- Easier interaction. Neutrons use the nuclear interaction (vs. electromagnetic interaction for X-rays, electrostatic interaction for electron beams, or polarizability for optical probes). Scattering by atomic nuclei results in detection of nearly all atoms.
- Good scattering for low Z elements. This is ideal for hydrogen- or deuteriumcontaining samples. Neutrons also have the ability of distinguishing between neighboring elements (e.g., Mn, Fe, Cr) and among different isotopes of an element.
- Isotopic substitution. The most common example is the substitution of hydrogen with deuterium in hydrogenous systems. Dependence of neutron scattering amplitude on isotopes of the same element allows the partial structure factors to be separated from the neutron diffraction data. This can then highlight the contribution of the substituted nuclei, revealing both structural and dynamical details of a sample without changing the chemical composition.
For many years, 239Pu samples were considered unsuitable for neutron scattering experiments because of their large absorption cross section (σabs = 1017 versus 18.5 barns for 239Pu and 242Pu respectively). Therefore, 242Pu samples were preferred. However, 242Pu is less relevant for long term response to stimuli and aging studies (many old samples are 239Pu), and more importantly, of lower availability than 239Pu. Since 2011, redesigned sample containers and a more appropriate sample geometry allowed us to pursue neutron scattering measurements at the Lujan Center using both diffraction (neutron) and a more challenging pair distribution function analysis using 239Pu samples, overcoming 242Pu availability issues.
Pu-Ga Alloys investigated by TOF neutron diffraction
Neutron diffraction measurements using two high-purity polycrystalline samples of δ-phase 239Pu stabilized with 2- and 7-at.%-Ga were conducted on the high pressure-preferred orientation (HIPPO) TOF diffractometer at the Lujan Center pulsed-neutron spallation source during the FY16, 17, and 19 run cycles (Figs. 1–4). In all three sets of experiments discussed here, samples underwent cooling from room temperature to cryogenic temperatures followed by a heating back to ambient.
The FY19 experimental series contains the most detailed data sets. For each cooling-heating sequence the material showed thermal expansion with a contraction of the lattice parameter upon cooling and expansion upon heating back to room temperature, consistent with previous results (Fig. 5). The final value of the lattice parameter measured upon return to room temperature was larger than the original starting value for each sequence, indicating that during each cooling-heating sequence the lattice expands beyond the initial state. This effect is most dramatic with the first sequence—it decreases progressively with each subsequent cooling-heating sequence and is almost negligible for the final one, suggesting that the effect is reaching a saturation level. There is also a gradual decrease in the difference between the lattice parameters measured at the same temperature during cooling and heating segments.
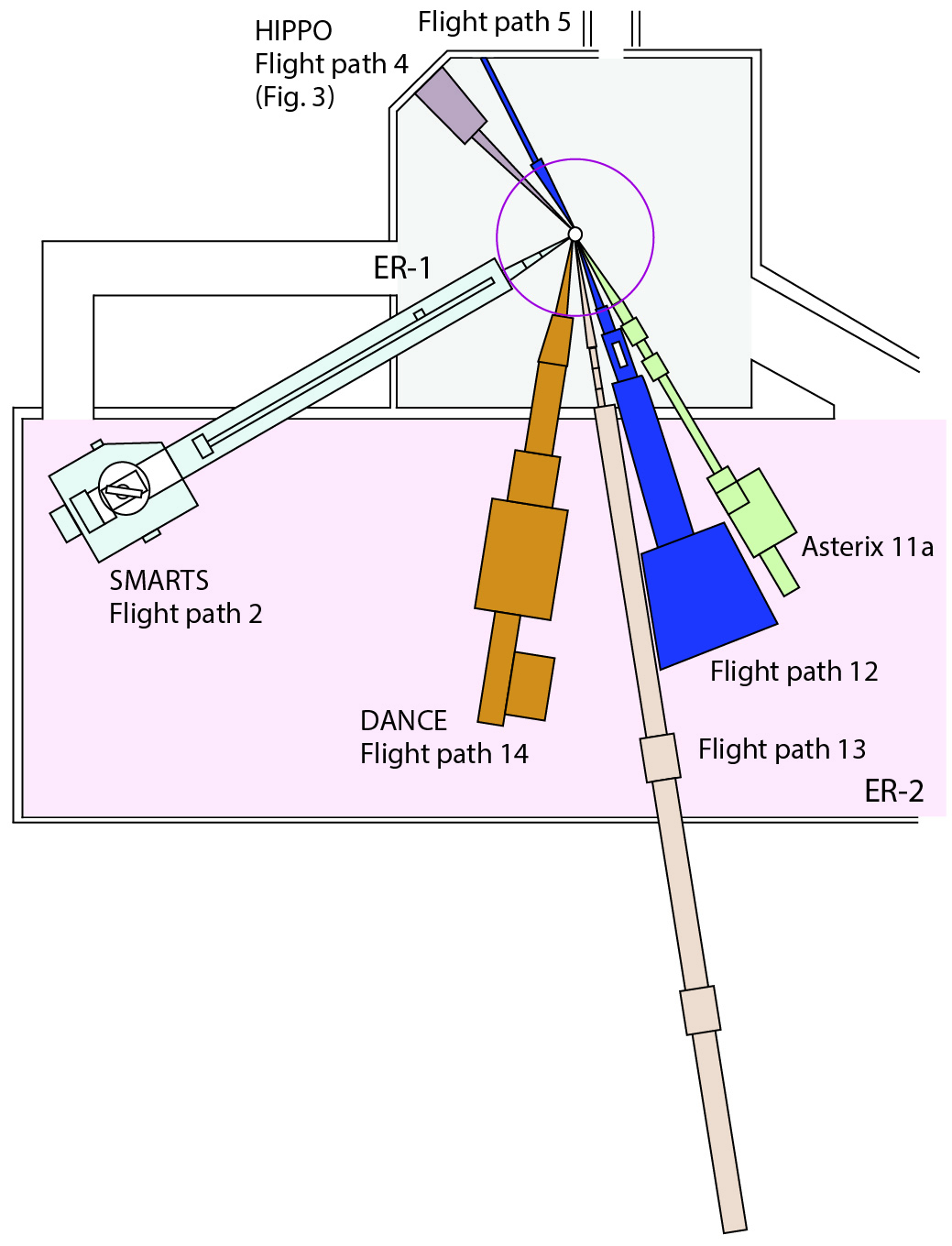
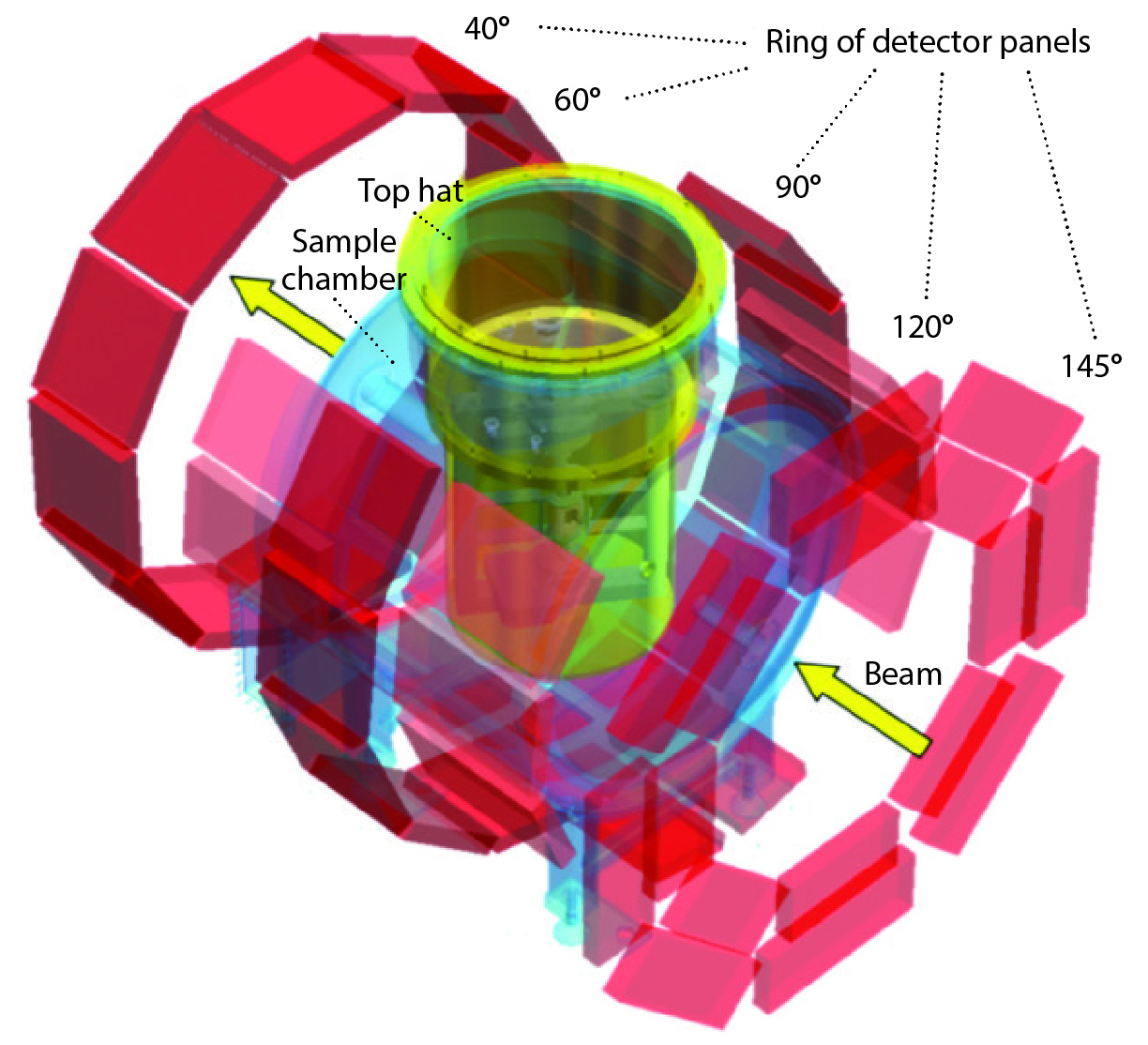
Similar behavior was observed in the FY17 experiment using the δ-phase 239Pu-2-at.%-Ga sample (Fig. 6a). However, the FY16 experiment using the same sample showed the opposite behavior, i.e., an overall contraction after being heated back to room temperature (Fig. 6b; as a reference, Fig. 6c shows the lattice parameter behavior as a function of temperature of the δ-phase 239Pu- 2at.%-Ga sample during the first cooling measurement of the FY19 run cycle experiment).
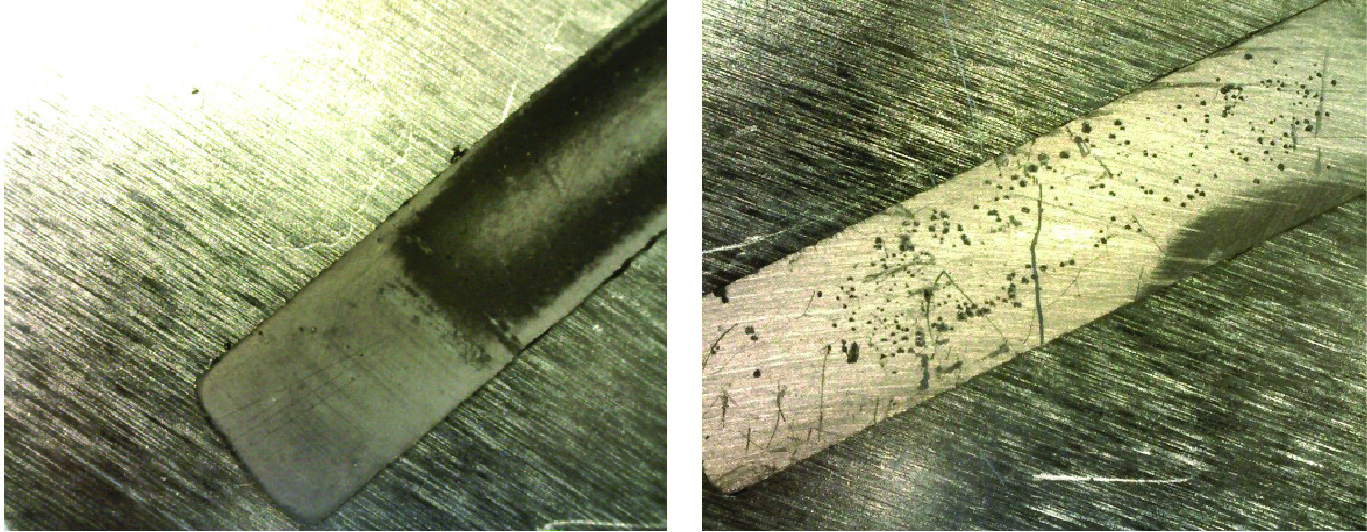
Idling time of samples
For a better comparison of the thermally-induced lattice swelling behavior (FY17/19 experiments) versus the contraction (FY16), we considered the thermal history of the sample and the (room temperature) storage time. The so-called “idling time” was defined as the period between sample annealing (i.e., reaching RT) and the beginning of a measurement.
For the FY17/19 experiments using the δ-phase 239Pu-2at.%-Ga sample, the annealing of the sample ended within a few hours of the start of the measurements, thus the sample can be considered fully annealed or “fresh”, and free of self-irradiation induced defects. For the FY16 experiment using the same sample, the measurement occurred a few months after the sample annealing was completed. This was due to unfortunate events related to sample shipping.
When annealing was performed within hours of the measurement, the final lattice parameter value was significantly larger than the initial value. The FY16 data set, for which annealing was completed a few months prior to the start of the experiment, reveals the opposite effect, namely an overall contraction of the lattice. For newly annealed samples, the exhibited lattice swelling is similar to that observed by Ravat et al. with isothermal holds of up to three years, reported in the Journal of Nuclear Materials in 2007. A saturation of this effect was observed by these researchers after two years of isothermal hold, whereas values reported here (FY19) achieve saturation by the fourth sequence. If the material is kept for a long idling time and later cooled to cryogenic temperatures then it shows minimal lattice swelling and an overall contraction upon return to RT. In summary, the kinetic processes of induced lattice contraction and lattice swelling behaviors proved to be dependent on the history of the sample.
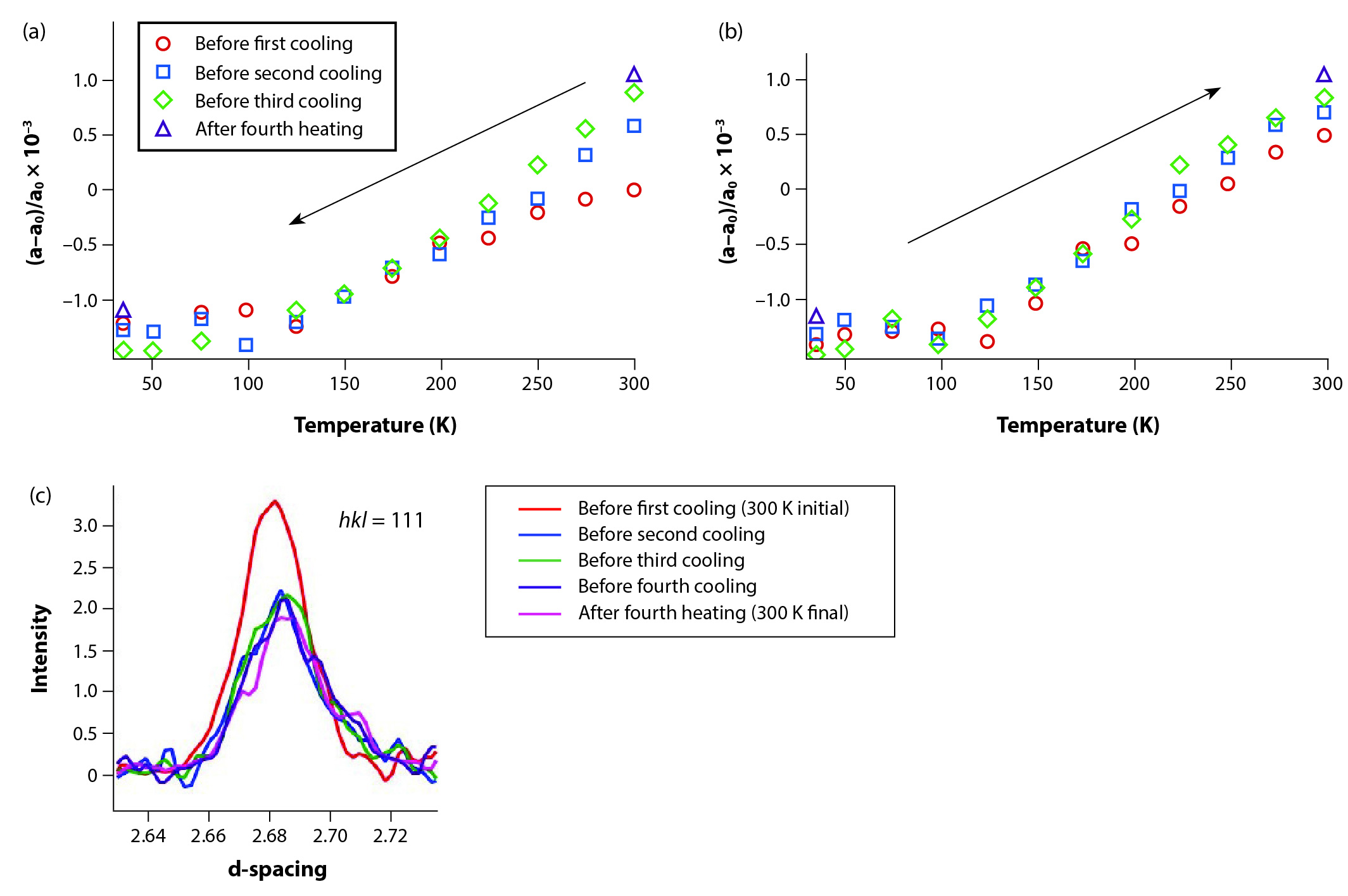
Similar experiments performed during FY16/17 on the δ-phase 239Pu-7at.%-Ga sample show comparable results in terms of overall contraction of the lattice when the idling times were significant, days to months (Figs. 6d,e). Therefore, the idling time effect appears to be the result of an incomplete recovery of the lattice parameters, and it has been observed for both δ-phase 239Pu-2 and -7at.%-Ga and samples.
In terms of peak shape, the effect translates into significant changes when a freshly annealed sample is cooled from ambient to cryogenic temperatures (FY17, 12K; FY19, 35K; Figs. 6a,d inserts). Upon heating and returning to ambient temperatures, the height of the peaks is not recovered, and it exhibited little change when the cooling/heating sequence was repeated (FY19). The peak positions shifted to lower d-spacings as the sample was cooled, but recovered upon heating back to room temperature. The loss of peak height and integrated intensity may indicate a loss of crystallinity and the potential of deformation mechanism under radiation induced hydrostatic stress increase.
We can conclude that the changes in peak shape between room temperature and cryogenic point are significant for a fresh sample, as observed for both FY17 and 19. Additionally, for the FY19 measurements, no significant variation of peak parameters was observed over the subsequent cooling/heating sequences (second → fourth). The response of the material to peak shifting and FWHM/integral intensity is due to either the thermal expansion under temperature change or to homogeneous strain, such as lattice response to hydrostatic stress due to irradiation changes. The response observed by Maiorov et al., reported in the Journal of Applied Physics in 2017, showed two stress components (kinetic adiabatic hydrostatic/adiabatic bulk elastic modulus and shear/shear elastic modulus) due to changes induced by self-irradiation. Ultimately, it is this idling time, during which the δ-239Pu lattice accumulates point defects and kinetic factors control a very complex point defect diffusion, along with an evolution process that cause extended defects or damage and voids.
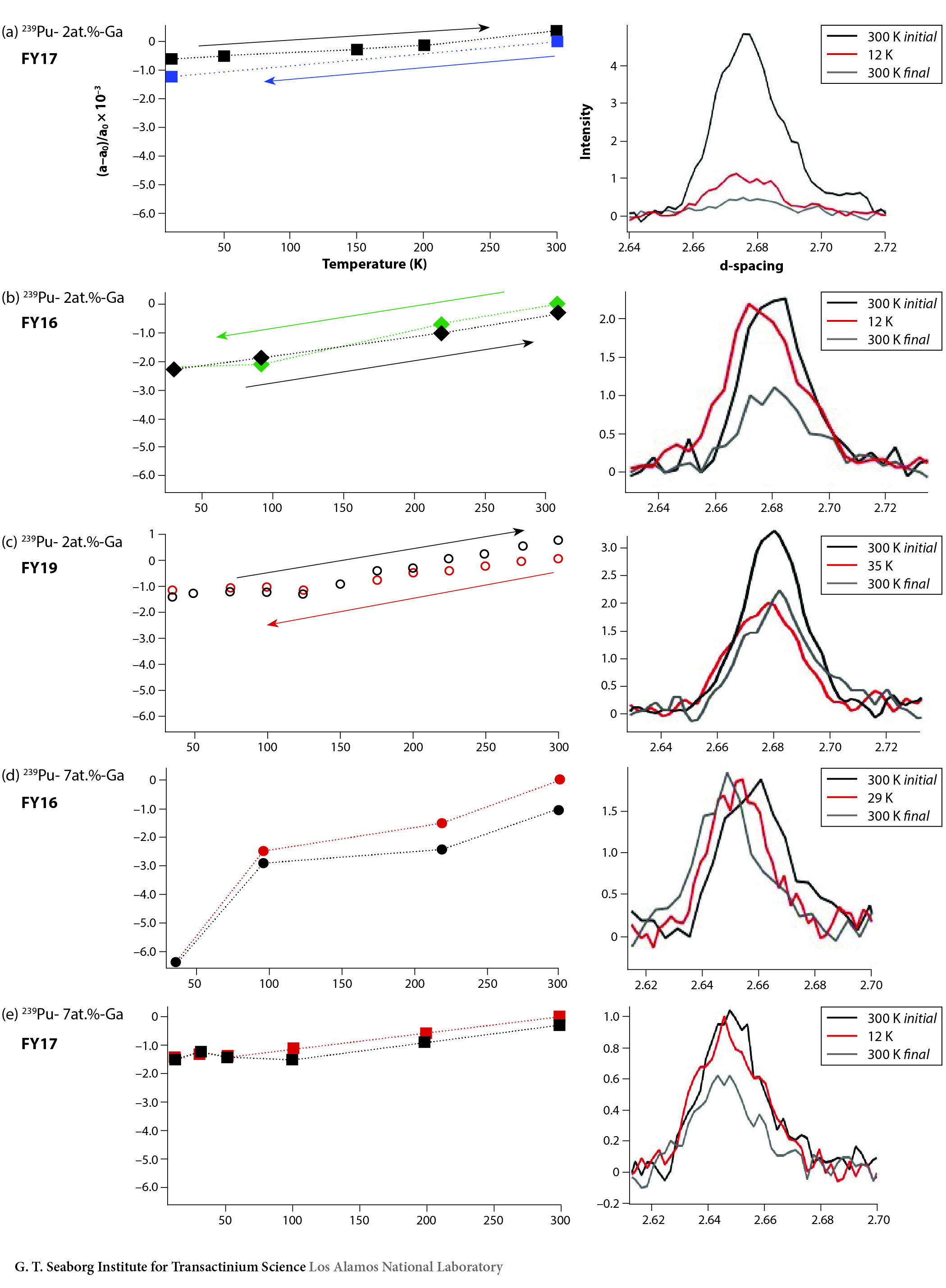
Long-range order
The most significant characteristic of this series of experiments is the impact on the long-range crystallographic order of these Pu-Ga alloy samples and on the kinetics associated with their varied thermal histories. In materials which are stable, the strain of thermal expansion stress which occurs with thermal cycling is observed and is repeatable. However, in these measurements of δ-phase 239Pu-Ga alloys, which are metastable and undergoing self-irradiation, we have to consider additional stresses from defects and defect evolution, exhibited in Pu as additional stress and correlated strain.
Diffraction peak shifting was observed due to homogeneous strain such as thermal expansion under temperature change or as a lattice response to hydrostatic pressure changes. A combination of these effects was observed that were correlated with both the thermal history of the sample and the potential for defects which could induce internal stress (defect-induced hydrostatic or shear pressure). Furthermore, we observed some evidence of loss in diffraction peak integrated intensity which is indicative of long-range order lattice deformation leading to loss of crystallinity.
The results are clearly affected by the ambient isothermal storage time during which self-irradiation induced defects and other changes arise in the δ-phase Pu-Ga lattice. These effects have proven to be complex and challenging to elucidate. As shown by a number of other studies employing X-ray diffraction and dilatometry, the self-irradiation effects converge into a time-dependent lattice swelling or strain in δ-phase 239Pu-Ga alloys. As a secondary observation, the bulk strain appears to impact the low temperature martensitic phase transformation δ → αʹ, preventing it from taking place. Earlier neutron diffraction measurements of δ-phase 242Pu-Ga alloys showed a hydrostatic and a shear component to the lattice strain that increases at cryogenic temperatures.
Summary
This study combines results from several experiments for a better understanding of the correlations among the complex thermal history, self-irradiation defects, and the kinetic processes associated with the evolution of long-range order damage in δ-phase Pu-Ga alloys. In-situ TOF neutron diffraction measurements were performed between room and cryogenic temperatures to explore the impact of ambient aging on the lattice effects. Specifically, those effects that were detectable during alternating temperature measurements on the lattice of a δ-phase 239Pu-2at.%-Ga alloy. Our results showed that the self-irradiation damage at room temperature and the associated lattice strain introduce a hydrostatic stress state in δ-phase 239Pu-Ga alloys. This impacts the kinetic evolution of defects which are generated from self-irradiation at low or cryogenic temperatures, as they evolve during heating to ambient temperatures.
Furthermore, in annealed δ-phase 239Pu-Ga alloys which were exposed to repeated cooling and heating (i.e., cryogenic self-irradiation followed by annealing to ambient temperatures) we observed a lattice swelling similar to the room temperature self-irradiation damage accumulated over longer periods of time. This point of extensive accumulation of self-irradiation defects at cryogenic temperatures and the kinetic evolution of those defects with increasing temperature is a driver to similar lattice strain states as the same material would achieve at ambient temperature.
Acknowledgments
The author would like to thank her collaborators who contributed to this work: Franz J. Freibert (MST-16/NSEC), Jianzhong Zhang and Sven Vogel (HIPPO, Lujan Center/MST-8), Joan E. Siewenie (P-27), Scott Richmond, Mike Ramos and James Gallegos (sample preparation, MST-16).
This work was supported by the US Department of Energy through the Los Alamos National Laboratory Science Primary Assessment Technologies (Campaign 1). The FY17 work was partially funded under 20150057DR “Aging in Delta Plutonium Alloys: A Fundamental Approach" LDRD-DR project. Los Alamos National Laboratory is operated by Triad, LLC, for the National Nuclear Security Administration of the US Department of Energy (contract DEAC52-06NA25396).
Alice Smith
Alice Smith joined Los Alamos National Laboratory as a postdoctoral research associate at the Lujan Neutron Scattering Center, and is currently a Staff Scientist in the Materials Science and Technology Division, Nuclear Materials Science (MST-16) group. Her research interests are focused on actinide science, with emphasis on plutonium science: self-irradiation damage and aging, thermodynamic properties and kinetics of phase transformations, and microstructure and defect formation of plutonium and its alloys.
Further reading:
- S.S. Hecker, “Plutonium—An element never at equilibrium,” Metall. Mater. Trans. A, 2008, 39 (7), 1585.
- W.G. Wolfer, “Radiation effects in plutonium,” Los Alamos Science: Challenges in Plutonium Science, N.G. Cooper, J.A. Schecker, Eds., Los Alamos National Laboratory, Los Alamos, NM, 2000, 26, 274.
- A.C. Lawson, J.A. Roberts, B. Martinez, R.B. Von Dreele, B. Storey, H.T. Hawkins, M. Ramos, F.G. Hampel, C.C. Davis, R.A. Pereyra, J.N. Mitchell, F. Freibert, S.M. Valone, T.N. Claytor, D.A. Viskoe, F.W. Schonfeld, “Lattice constants and anisotropic microstrain at low temperature in 242Pu–Ga alloys,” Phil. Mag., 2005, 85 (18), 2007.
- B. Ravat, B. Oudot, N. Baclet, “Study by XRD of the lattice swelling of PuGa alloys induced by self-irradiation,” J. Nucl. Mater., 2007, 366 (1–2), 288.
- B. Maiorov, J.B. Betts, P. Söderlind, A. Landa, S.C. Hernandez, T.A. Saleh, F.J. Freibert, A. Migliori, “Elastic moduli of δ-Pu-239 reveal aging in real time,” J. Appl. Phys., 2017, 121 (12), 125107.
- A.I. Smith, K.L. Page, J.E. Siewenie, A.S. Losko, S.C. Vogel, O.A. Gourdon, S. Richmond, T.A. Saleh, M. Ramos, D.S. Schwartz, “Time-dependent local and average structural evolution of δ-phase 239Pu-Ga alloys,” MRS Adv., 2016, 1 (44), 3019.
- A.I. Smith, A.S. Losko, S.C. Vogel, S. Richmond, M. Ramos, Plutonium Futures—The Science 2016, Proceedings, Baden- Baden Germany, 2016, 260.
- A.I. Smith, S.C. Vogel, J. Zhang, A.S. Losko, T.A. Saleh, S. Richmond, M. Ramos, J. Gallegos, F.J. Freibert, Plutonium Futures—The Science 2018, Proceedings, San Diego CA, 2018, 39.
- A.I. Smith, “Diffraction and pair distribution function analysis,” in Plutonium Handbook, Second Ed., 2019, 6 (43), American Nuclear Society.
- S.C. Hernandez, F.J. Freibert, J.M. Wills, “Density functional theory study of defects in unalloyed δ-Pu,” Scr. Mater., 2017, 134, 57.