When Sanna Sevanto came to Los Alamos in 2009 to study tree mortality in the desert Southwest, she was surprised by the landscape. “Why is tree mortality patchy?” Sevanto recalls thinking when she first looked at the hillside—some trees were dead and others were fine. Blocking the intense sun from her eyes as she turns to the Sangre de Cristo mountains, Sevanto explains that one of the reasons behind this patchiness could be the microbes in the ground, the soil microbiome. “The microbiome community beneath one tree can be very different than that beneath a tree 10 feet away,” she explains. “And the microbes contribute to the health of the trees.”
Sevanto did not grow up as a farmer, or with a particularly green thumb. But as a physicist with expertise in atmospheric sciences and ecosystems, she was drawn to the study of tree mortality. Now, along with several other scientists at Los Alamos, Sevanto is looking to the soil for clues about how to grow healthy trees and plants for food and energy. The question is simple: all things being equal, how is the soil beneath a healthy tree different from the soil beneath an unhealthy tree?
Plants, like humans, need a healthy microbiome. The microbiome of each tree in the forest is influenced by many factors, such as how many dead plants and animals are decomposing nearby or whether the contours of the land allow extra water, leaves, or needles to gather at the tree’s base. Any of these factors can influence the specific microbiome of a plant, just as a human’s diet, lifestyle, and travel habits influence the microbiome of their gut.
Sevanto and her Los Alamos colleagues want to know specifics: which microbes are helpful and how can we keep them flourishing? This task is not trivial. Although scientists have long known about our coexistence with microorganisms, the focus for centuries was on the ones that cause disease. Only in the last few decades—and through the use of DNA and RNA sequencing—have scientists discovered that there is a vast diversity of microorganisms that contribute positively to the health of our bodies and the planet. But the specifics about precisely which microbes are good, and in what ways, have been especially elusive.
Los Alamos scientists are digging in. With long-standing expertise in genetic sequencing, custom bioinformatics tools, and interdisciplinary approaches, Lab scientists are investigating the role of microbiomes on plant productivity, particularly with respect to the security of the nation’s food and fuel. Across the globe, scientists are predicting a warmer and drier future which could make it challenging to grow plants for food and energy, as well as to sustain forests and Earth's carbon balance. There are many aspects to this problem, but some of the solutions might already be in the soil.
Microbes, they get the job done
Humans have been cultivating plants for thousands of years, and they’ve learned the basic requirements: sunlight, water, carbon dioxide, and nutrients such as nitrogen. Ancient farmers may not have known yet about chemical elements, but they figured out that using manure and planting legumes near their crops increased yield—both practices are now understood to help add nitrogen to the soil. In fact, before nitrogen was officially discovered in 1772, an Englishman named John Evelyn had already noted in 1675 that something beneficial existed in rainwater, and he called it “celestial nitre.” By the 1950s, farmers had begun to use synthetic nitrogen fertilizer, and this change facilitated huge advances towards the industrial agriculture that humanity relies on today.
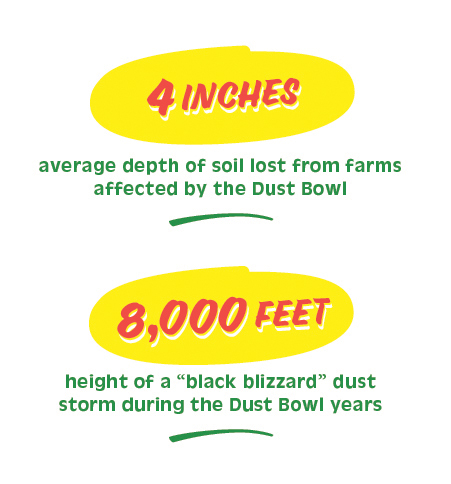
But natural ecosystems provide their own fertilizer. Plants can’t use atmospheric nitrogen because in its molecular form (N2), its triple bond is too strong to break. However, microbes in the soil and water can do it: they digest N2 and convert it to forms that plants can use, such as ammonium (NH4+), nitrite (NO2-), or nitrate (NO3-). Microbes also break down and decompose dead plants and animals, releasing their nitrogen and other nutrients like phosphorus, zinc, copper, and iron. These nutrients are essential for plants to thrive. Nitrogen is especially important for photosynthesis, and phosphorus is vital for many processes such as photosynthesis, sugar metabolism, and growth. To complete the partnership, the plants excrete certain sugars into the soil, to feed the microbes.
The physical nature of soil is a complex mixture of living things—bugs, microbes, plant roots—as well as decomposing organic matter. “Organic matter is fluffy and provides pathways for water to get deep into the ground instead of running off the surface,” explains Sevanto. Tilling and plowing soil for agricultural planting disrupts the structure of organic matter. The process breaks roots and filamentous fungi and introduces air to areas usually hidden underground. Although tilling reduces weeds and kills pathogens (many of which are fungi), if it is done too deeply or frequently, it can disrupt certain soil ecosystems; damaging the physical structure of the dirt can dry it out, killing not only dangerous microbes, but also beneficial ones.
“Organic matter is fluffy and makes pathways for water to get deep into the ground instead of running off the surface.”
Without a network of living roots and fungi, the soil becomes compact, leading to more runoff and less water infiltration. This leads to further deterioration: compacted soil is more difficult to plant, leading to more tillage, plus fewer microbes leads to fewer nutrients, so farmers must add more synthetic fertilizers. Synthetic fertilizers help feed millions, but they're also made from fossil fuels and, when overused, can leach into waterways and damage surrounding ecoystems.
After the Dust Bowl of the 1930s, soil scientist Hugh Bennett guided some of the Roosevelt administration’s response to the devastation. Bennett had studied soil types and observed differences between the rich, black soil in the forests and the dry, crumbly soil in dessicated farms. He helped educate farmers about using cover crops between their plant rows to add and maintain soil structure, and about changing their plowing practices to capture more rainwater by contouring the land. Bennett, who founded the Soil Conservation Service, now called the Natural Resources Conservation Service, knew that through science and education, farmers could improve their relationship with the soil. Like Bennett, scientists today know that healthy soil is key to sustaining farmland—but they also know there is more to soil science than meets the eye, and there is still much to learn.
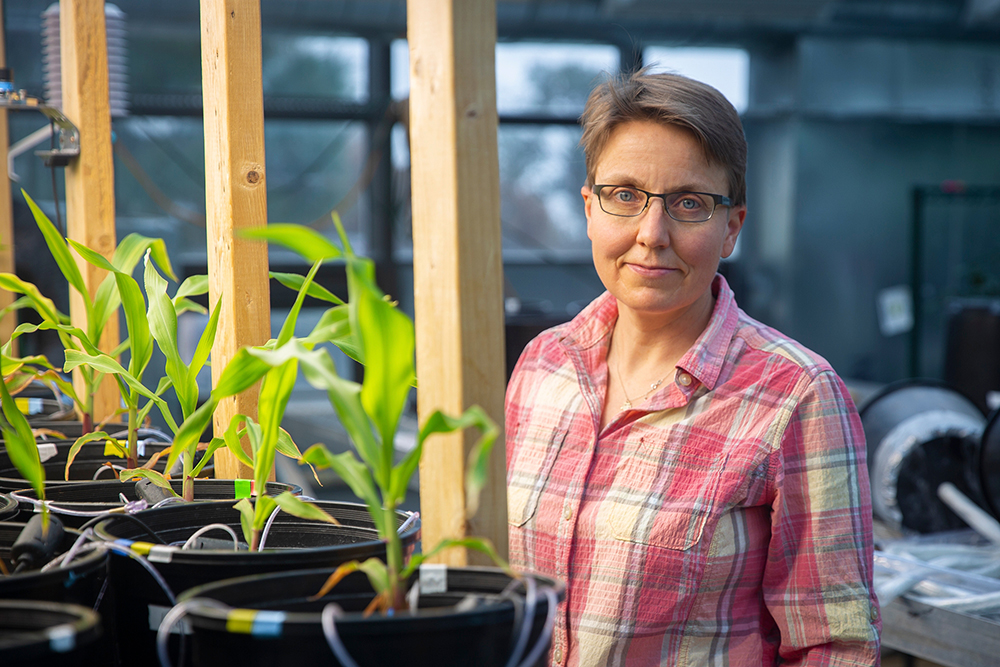
“We just don’t really know what normal is with soil,” says bioinformaticist and Laboratory Fellow Patrick Chain, who leads a multi-lab project to understand bacterial and fungal relationships in soil. Soil is, quite literally, a black box—millions of microbes that are invisible to the naked eye, but doing something important. Chain explains that over the last 20 years, scientists have been able to sequence the DNA from many soil microbes and have learned about some of their individual functions. However, soil is a complex and heterogenous environment, and there is much that is unknown and difficult to decipher, such as how microbes interact with each other in various types of soil habitats, or niches. In their natural environment, microbes can sense and respond to other mutualistic microbes that may help them, or to pathogenic microbes that may inhibit or threaten their survival. When taken away from their natural environment and into a lab, many soil microbes cannot grow in isolation, and even when they can, the microbes’ behavior may be different without their co-habitants.
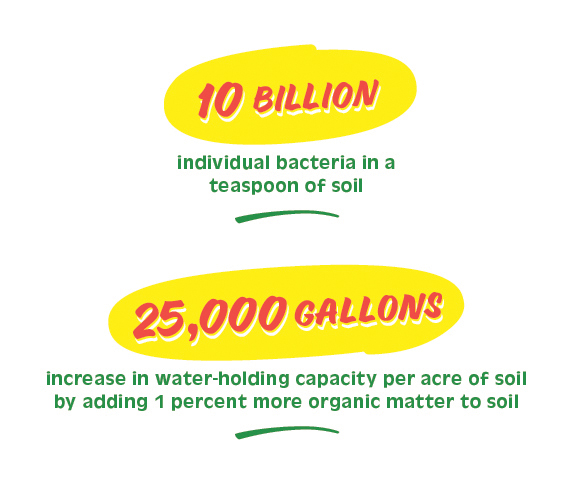
Unfazed by the black-box challenge, Chain, Sevanto, and several other scientists at the Lab are each beginning to unearth the secrets of which microbial relationships help plants thrive, especially in extreme heat and drought. The scientists are exploring new approaches and combining efforts with others—and all are driven to learn more about the soil to help humans adapt and secure the future of our ecosystem.
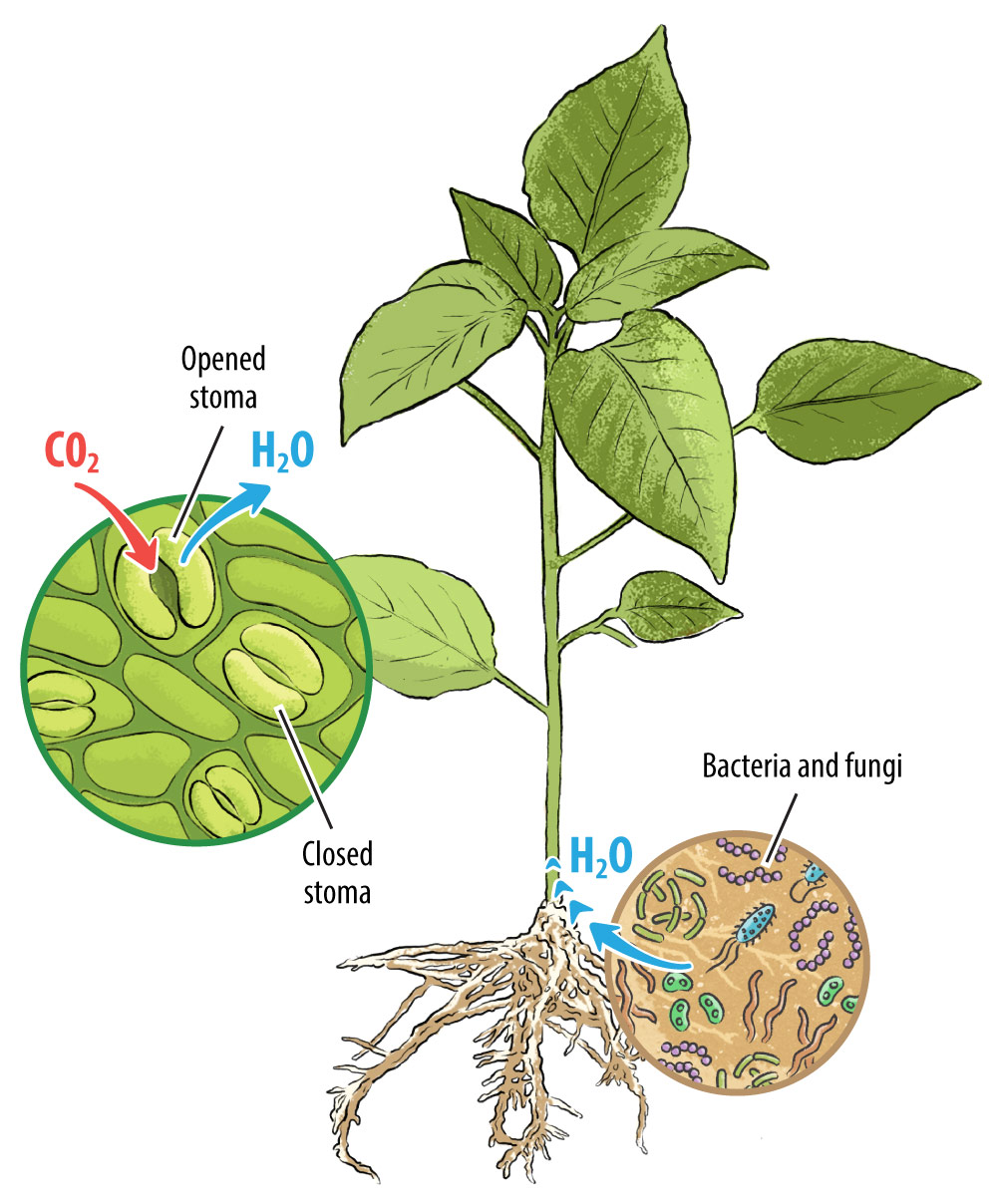
It takes a village
Plants have multiple strategies to adapt to drought—many of which involve limiting growth. For example, stomata are microscopic pores found in plant leaves and stems, that operate like a mouth for the plant and participate in growth regulation. When stomata are open, the plant can “eat” valuable carbon dioxide needed for photosynthesis to create sugars for energy and the growing of new leaves, flowers, and fruit. However, also when the stomata are open, water vapor escapes from the plant, which can lead to dehydration. For this reason, plants generally keep their stomata closed when it’s hot or when water is scarce. In an effort to reduce water loss, the plant sacrifices potential growth by limiting access to carbon.
Scientific studies of stomata closure and other growth-limiting functions, in pursuit of creating drought-resistant plants, have often focused on the genes that control these functions. Sevanto’s team, however, may have found a different approach to improving productivity and drought tolerance that does not involve the plant’s genes but instead, its microbiome.
Sevanto and her colleagues compared two groups of pinyon pine trees: one group was known to have genes that made the trees drought tolerant, while the other group of trees was known to be intolerant. The scientists inoculated seeds from each group with a root-associated fungus to study its impact on tree growth. The team measured the trees’ water uptake, belowground biomass, root length, and stomatal closure point (the temperature at which stomata will close to conserve water)—and their results were somewhat surprising.
When the fungus was present, water uptake was improved for some trees and they kept their stomata open longer, continuing photosynthesis even in drier conditions. “This study shows, for the first time, that the stomatal closure point can be affected by plant-microbe interactions,” says Sevanto. In other words, all other things equal, the makeup of the microbiome can influence a plant’s functions.
Another interesting result from the pinyon study was that the fungus affected each group differently. It helped the drought-tolerant trees but was detrimental to the other trees. This suggests that plant-microbe interactions can be more than just species-specific: microbes that are helpful to one group may not be helpful to another group within the same plant species.
Because microbiomes are so specific, different plants rely on different signals to trigger stomatal closure. However, Sevanto explains that if they can identify the right microbe-plant combinations, they could use the microbes to help plants maintain their productivity with less irrigation.
With the right microbes, plants can maintain their productivity with less irrigation.
Excited by these data highlighting the importance of the microbiome, Sevanto’s team conducted several more studies, asking: Which microbes are most helpful for drought tolerance in various species? How effectively can a microbiome change in response to environmental conditions?
“Many studies show that plants and microbes talk to each other. Plants can attract microbes based on what they need to thrive. For instance, plants exude specific sugars to attract microbes that may help them get and retain water,” says Sevanto. “We hypothesized that if we grew a plant in dry conditions, it would likely attract a microbiome that would help it survive.”
To test this, Sevanto’s team grew maize plants with microbiomes from two different soils—one from an agricultural field in Colorado and the other from a pine forest in New Mexico. The scientists planted the maize in small pots of artificial soil and then added a small amount of either the agricultural soil or the forest soil. They gave ample water to half of the plants in each group, and limited the water for the rest. The scientists used genetic material from the test soils to determine which microbial species were present and abundant.
The team found significant microbiome differences between the two soils, and they also found differences in how the microbiome communities changed in response to water availability. Sevanto’s team wanted to drill down into the differences they found, so they next developed a way to direct the evolution of a drought-tolerant microbiome. The team took soil from the best-performing plants from each generation of the forest soil group and the agricultural soil group, and gave it to the next generation of plants. The results of this study are still being analyzed, but preliminary data support the initial assertion that the microbiome composition can influence how plants function under changing water availability. Going forward, this premise could help improve plant productivity for food, biofuels, and soil carbon sequestration, by using microbes instead of genetic modification.
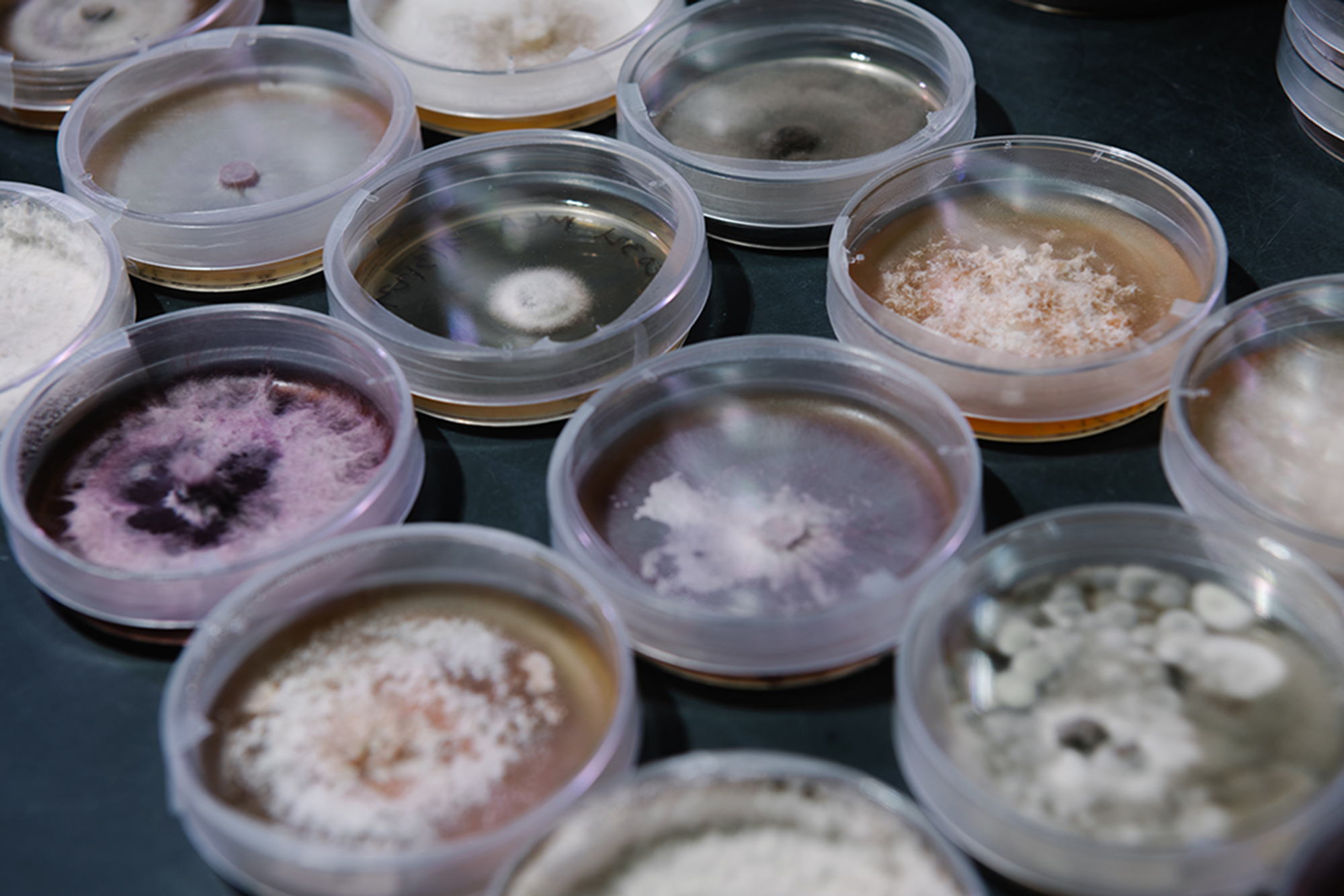
Leaders of the village
Los Alamos microbiologist Buck Hanson knows that not every soil bacterium or fungus can be isolated in the lab. But with a lot of effort and a little finesse, some of them can be, as evidenced by the stacks of petri dishes on Hanson’s lab benches. He has successfully isolated 300 fungi and 300 bacteria for a project about bacterial and fungal interactions. This project is part of a large Department of Energy, Science Focus Area (SFA) program on bacterial and fungal relationships, being led by Chain and his Los Alamos colleague Aaron Robinson.
In a somewhat opposite approach to Sevanto’s project, which aimed to direct the evolution of an entire microbiome that would be helpful during drought conditions, the SFA team chose to focus on an organism that is already adapted to such conditions—a drought-tolerant grass pervasive throughout the Southwest United States, called blue grama. Hanson and the SFA team are taking apart and analyzing one by one the microbial contents of blue grama’s microbiome.
“We want to understand what makes blue grama grass thrive in the arid Southwest. This knowledge could benefit bioenergy and agriculture crops,” says Hanson.
“We want to understand what makes blue grama grass thrive in the arid Southwest. This knowledge could benefit bioenergy and agriculture crops."
Hanson and his colleagues are studying soil from three distinct sites in New Mexico where blue grama grows. The first site is a transitional biome in central New Mexico at the Sevilleta Long Term Ecological Research site (elevation 1511 m, average annual temperature 14ºC, annual precipitation 2 cm), the second is on one of the mesas at Los Alamos (2231 m, 12ºC, 30 cm), and the third is in the Jemez Mountains at the Valles Caldera National Preserve (2466 m, 6ºC, 59 cm). Because blue grama grows at each of these locations—despite significant differences in elevation, annual temperature, and rainfall—the scientists hope to discover which microbial relationships are key in helping the grass adapt.
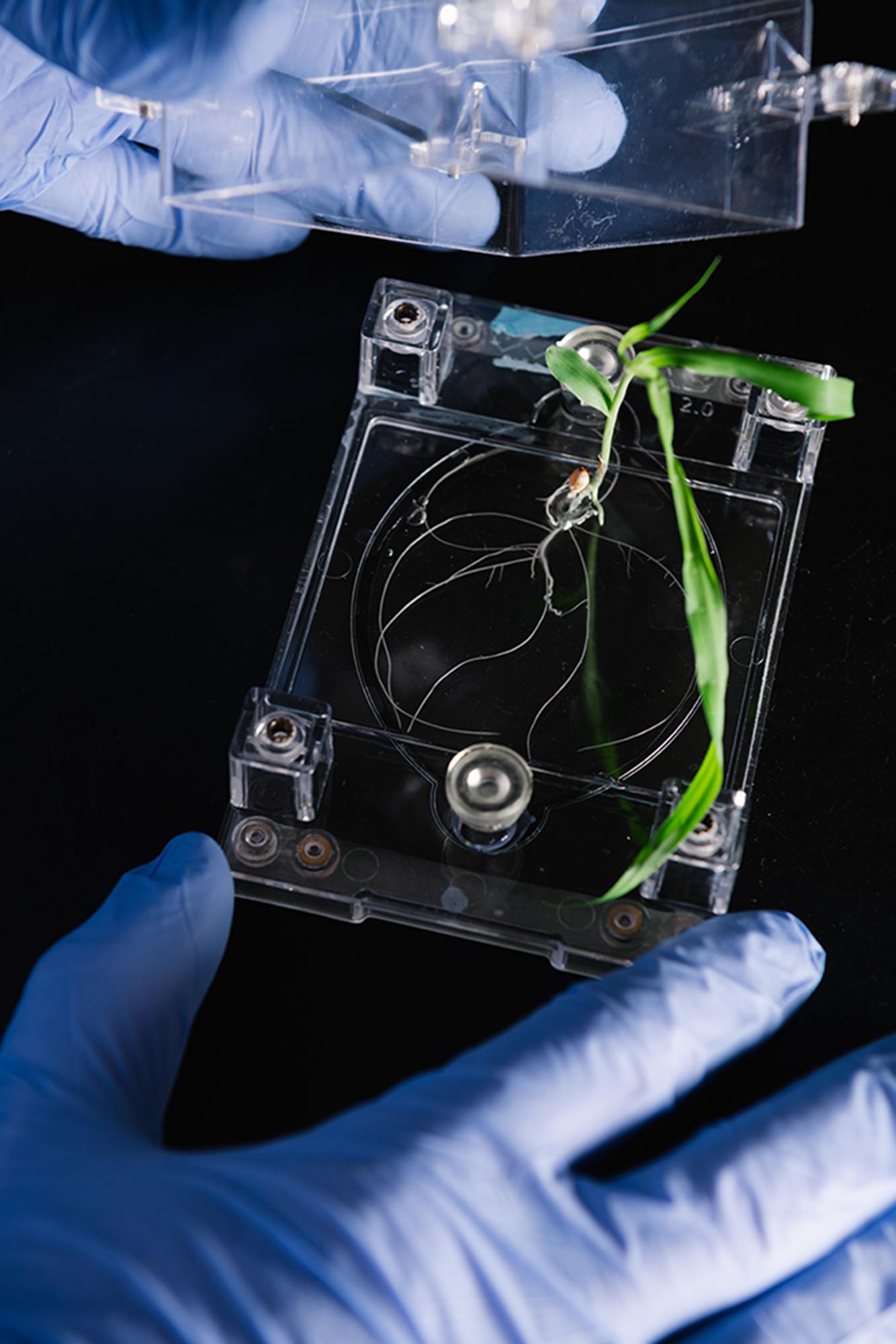
Hanson has grown his 600 isolates of bacteria and fungi from the soil at these three sites, each in its own petri dish, with carefully adjusted nutrients. The team is sequencing the genome of each isolate to compare to other known organisms. The researchers conduct metabolic tests that can tell them what functions are taking place, like whether or not a microbe of interest metabolizes nitrogen. Hanson and his colleagues are also using small growth chambers, called EcoFABs, to grow single sprouts of blue grama grass in sterile water, so they can add the microbes one at a time to study their effect.
“We can observe which microbes colonize around the root systems. We can also study interactions by identifying which genes are being turned on when microbes are together. For instance, if we add bacteria A and fungus B, we might be able to tell if they dislike each other, or cooperate with each other, by studying which genes are activated in each one,” says Robinson. It is important to remember that microbes live in mixed communities—some are friends and some are foes, and some are beneficial to the plants and some are not. To further complicate matters, the beneficial or harmful impact of specific microbes may differ depending on external environmental parameters. Identifying the functions of each microbe is a critical step in figuring out this balance.
Another key aspect of the bacterial-fungal interactions project is understanding the spatial relationships between the microbes. Many fungi are filamentous, meaning that they grow in the form of long, thin threads and stretch out in the soil like tree branches. Where exactly, along each fungal branch, do bacteria tend to congregate? To visualize bacteria along the filamentous fungi, the Los Alamos scientists put fluorescent labels on some bacteria before adding them to the EcoFAB sprouts. Then, using specialized microscopy to see the fluorescently labeled bacteria, they take photos for in-depth analysis. Team member and Los Alamos chemist Demosthenes (Dean) Morales also uses a technique called fluorescence in-situ hybridization (FISH) combined with a laser scanning confocal microscope to take images of labeled microbes that are living inside the fungi.
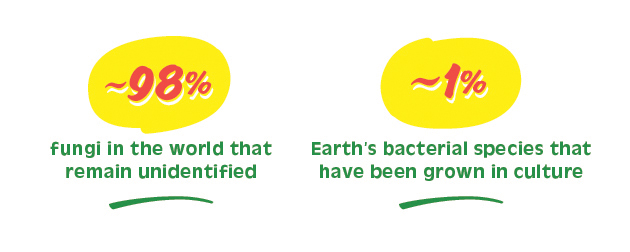
Using FISH, the team visualized bacteria that grow inside fungi, but they also found other bacteria on the outside, corroborating the idea that bacteria can travel along fungal filaments, using them like highways through the fluffy dirt. “In addition to imaging, our team is engineering tools to identify these functional interactions,” says Morales. “To learn more about bacteria that use fungi as highways, we implanted 3D-printed devices, shaped as hourglasses, into soil to isolate fungi that can grow vertically within the device towards a nutrient bait on the other end. We then identified bacteria that grew on the bait, suggesting that the bacteria used the fungi for transportation, since bacteria typically require a liquid medium to swim around.”
Overall, Robinson says that the vast majority of fungi in the soil are completely undescribed because they are difficult to culture in the lab—most laboratory culture techniques have been optimized for bacteria. Furthermore, fungal genomes are typically larger and more complex than bacterial genomes, making them more expensive to sequence, so fewer fungi have been fully studied. Learning about fungi, however, is critical to a more complete understanding of the soil ecosystem. “Because we’re dealing with so many unknowns in the soil, our team is focused on identifying core processes that might help us understand other microbes that we can’t isolate or grow in the lab,” says Robinson.
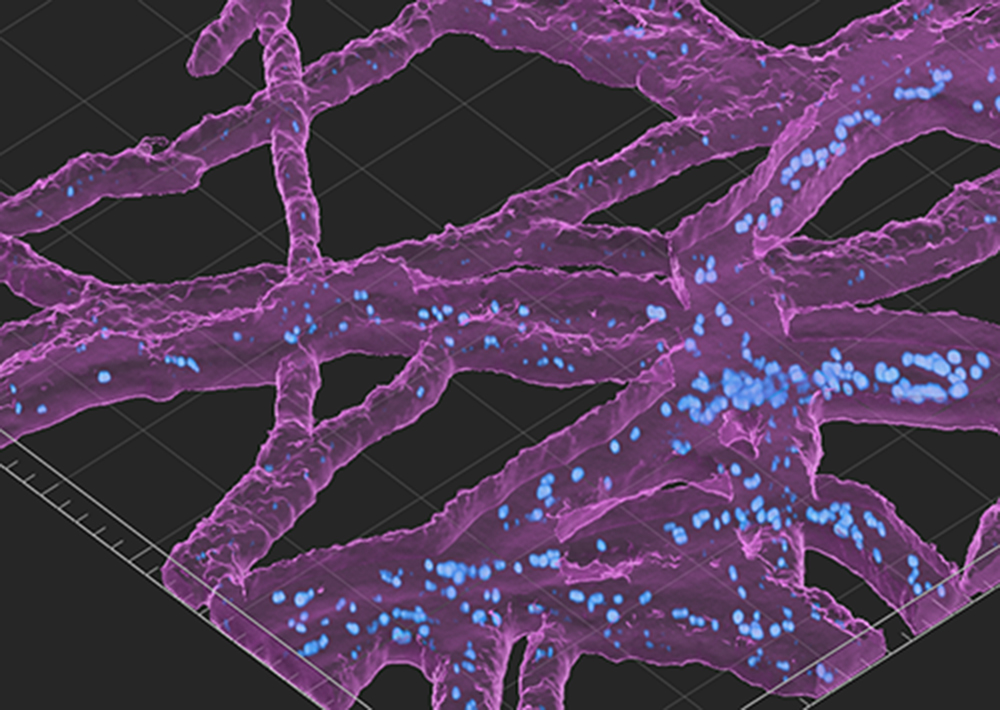
No stone unturned
Growing plants in a changing climate is a challenge. Scientists are showing that the soil microbiome is a critical component of any strategy, but they are also revealing that it is complex and highly specific to different plants and environments. There is still much more work to be done. These projects are just a few examples of how Los Alamos is tackling the problem, and new studies are currently underway to do even more. For instance, Hanson is leading an effort to more deeply understand how plants communicate with microbes using chemical signals. Sevanto is leading a project to investigate the benefits of adding carbon to soil using biochar (a charcoal-like material) and another project to study how microbes in plants and soils produce nitrous oxide—a greenhouse gas—when soil has been over-fertilized with too much nitrogen.
Because there is so much to learn about soil microbiomes, collecting and sharing data is critical to advancing knowledge. Members of the Los Alamos bacterial-fungal team are part of an important collaboration, the National Microbiome Data Collaborative (NMDC), to do just that. The NMDC maintains a portal for microbiome data and, true to its mission, ensures that the datasets are findable, accessible, interoperable, and reusable. Part of this mission also includes educating scientists on how to collect data in a standardized way, making datasets more usable for others.
“Allowing access to soil microbiome data is valuable and enables larger scale studies. This is super important for understanding the impact of microbiomes on carbon sequestration, soil health, plant health, and food security,” says Julia Kelliher, who is the NMDC Engagement team lead. “These data should not be limited to just one lab. The changing climate is something that impacts everyone and we want to make it possible for anyone to both find, and be able to re-use microbiome data in a meaningful way.”
The science of growing plants is the same as it always has been: sunlight, water, carbon, and nutrients. But today, more than ever, scientists are learning how nature works and adapts. These principles could be key to our success. Humans are going to continue to face challenges to finding hospitable farmland—and these challenges will only be exacerbated by the increased needs of a growing population. Nature has a lot of solutions, we just have to dig deep to find them.
With a Little Help from Their Friends
Los Alamos biologist Sangeeta Negi knows how to help her plants through stressful times, by strengthening them from the inside. In fact, she has designed a probiotic solution of friendly bacteria to do just that. Negi has studied plant growth for more than 15 years—everything from biofuel-feedstock Camelina and model plant Arabidopsis to nutrient-rich sorghum—and her work has led to a collection of about 20 beneficial microbes that can colonize plants of all kinds. What’s unique is they’re all endophytes: microbes that live inside a plant’s leaves, stems, or roots.
Negi’s endophyte-based “probiotic,” now under a provisional patent, is useful for many types of plants. While other research shows that beneficial microbiome communities can vary significantly between species, Negi identified a handful of microbes that colonize a wide range of crops and help the plants respond to stressors like increased soil salinity.
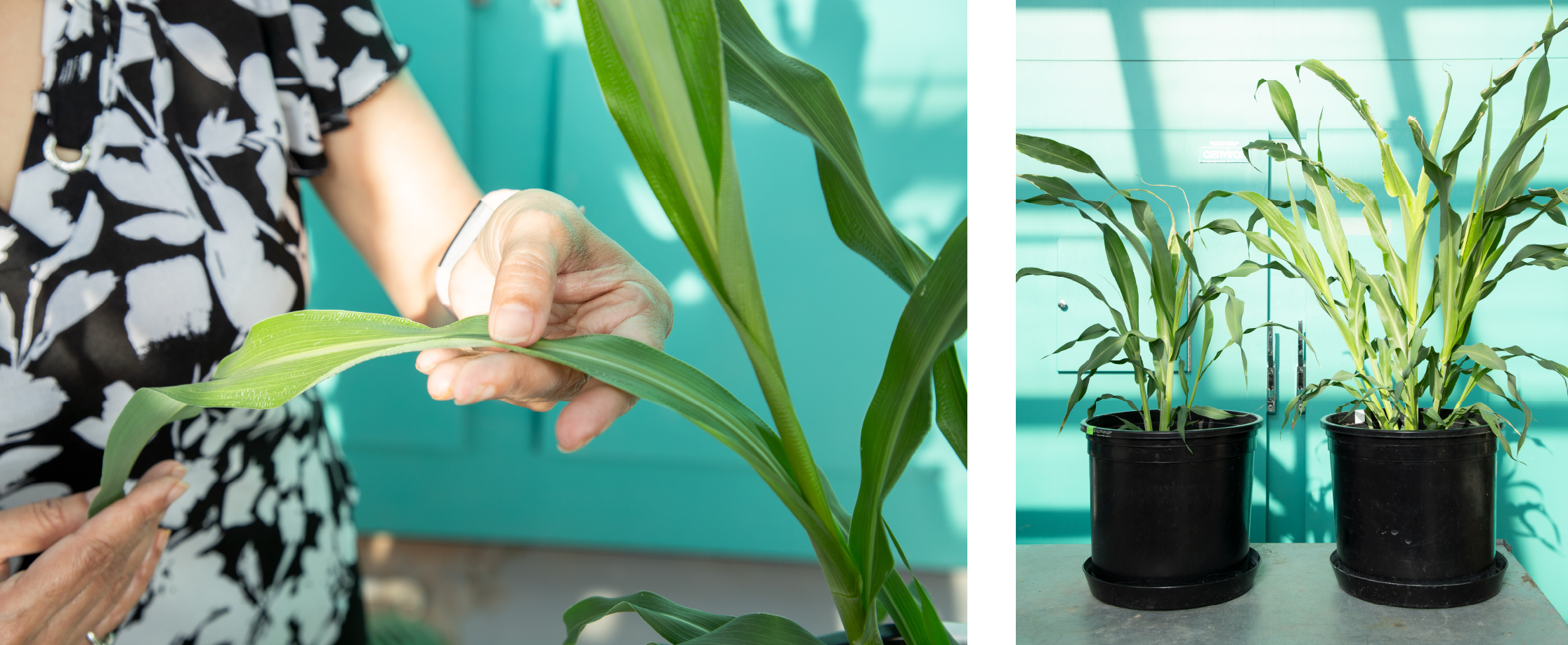
“One thing that happens during drought is that the reduced soil moisture leads to an increase in salt, which causes stress for plants,” says Negi. This increased soil salinity makes it difficult for plants to take in water—and under drought conditions there is already less water available. Plants sensing a lack of water will begin to release stress hormones that limit their growth and conserve resources. Negi’s approach mitigates this response. “We found a combination of microbes that help the plant by reducing stress hormone concentrations, boosting levels of other growth-promoting hormones and providing nitrogen and other nutrients,” continues Negi.
Negi and her team tested numerous mixtures of endophytes to determine the ideal combination that would be beneficial for many different plant varieties. They tested the best mix on camelina and sorghum under stressed conditions and found that it helps increase the plants’ resistance to stress. The probiotic bacteria mixture not only helped the plants survive in salty conditions, but also boosted growth. The treated plants showed longer roots with more robust branching, increased plant size, and more branches and leaves per plant compared to untreated plants.
Endophyte-based probiotics would give scientists and farmers an advantage. Other types of probiotics which might be sprayed on the outside of the plant are susceptible to the elements—frequent rainstorms—or even competition from other microbes in the environment. But endophytes living inside the plant are mostly protected from the harsh, exterior environment.
“Another advantage,” says Negi, “is that our endophytes can be inoculated into seeds before planting, and because of the bacteria’s close association with the leaves and stems as the plant grows, the bacteria are present when the plant makes new seeds, so the probiotics can be inherited by the next generation.” Long-term friends that help in times of stress—what more could a plant need?