While this reputation is surely justified in part, it is but one aspect of this anarchically temporal and almost paradoxical metal. Despite being an element that was born in wartime, and controversially used to help end that conflict, it has also found peaceful use as a source of both nuclear and thermoelectric power, in turn enabling the exploration of the solar system. From the orbits of an astronomical scale to those of the subatomic, we have also gained an incredible opportunity to peer into the deep complexities of the atom and the myriad competing factors that determine its properties, many of which are still a mystery to us.
Albert Migliori, former director of the Seaborg Institute in Los Alamos has said that “it is not unreasonable to consider plutonium as the most interesting element after helium in its challenge to our understanding.” An element that has been called a physicist’s dream but an engineer’s nightmare, it can change density by as much as 25% with little provocation. It actually increases in density when it melts and it is a poor conductor of heat or electricity—and the list of anomalies goes on and on. At a basic level, this is because of its location in the periodic table, at a tipping point between localized and delocalized electronic configurations. These properties are, however, almost entirely disconnected from the nuclear properties which plutonium is more widely valued for. As such, the study of plutonium is an inherently interdisciplinary activity.
In this article, I attempt to shine a light on this fascinating element with particular focus on the unique story of its discovery and its strange properties that continue to challenge our best theories.
Discovery: Hesperium, Extremium, or Plutonium?
Element 94 was first isolated in minute quantities in 1941 by Glenn Seaborg, Edwin McMillan, Joseph Kennedy, and Arthur Wahl at the Radiation Laboratory of the University of California, Berkeley. Yet the details of this momentous discovery weren't shared with the rest of the scientific community until 1946, after World War II—the only time a fundamental scientific discovery of this importance has ever been kept secret. The ink outlining the concept of a top-secret uranium bomb was still wet on the page, but in this tangential effort, they had almost immediately found a superior element for its fissionable component.
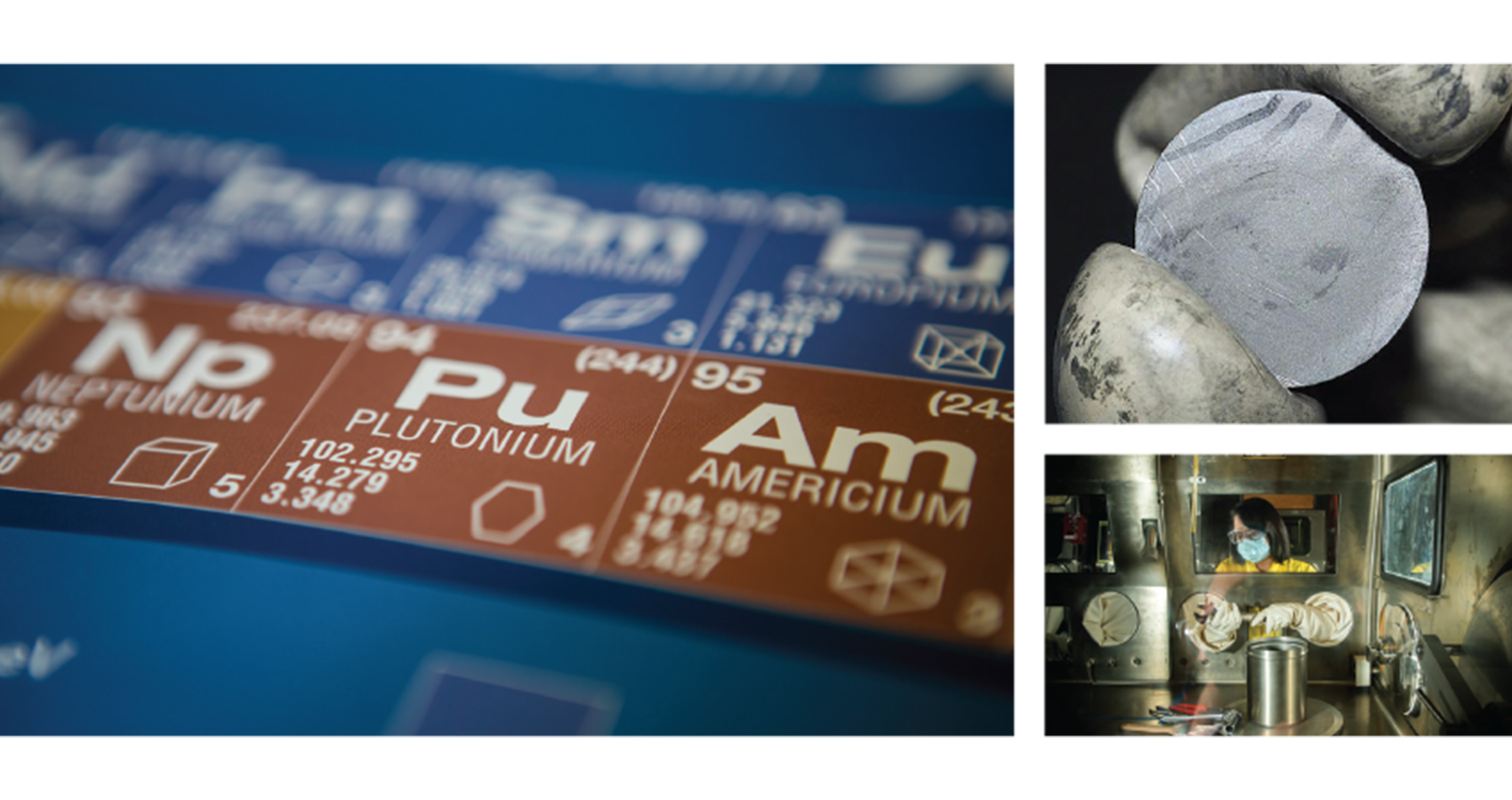
How did this come to be?
The discovery of plutonium is inexorably entwined with the development of nuclear fission, i.e., the splitting of the atom. Surprisingly, in only the decade before, some of the greatest scientists of the era, including ones that were directly responsible for the creation of the field, still held deeply pessimistic views on the prospect of nuclear fission:
“There is not the slightest indication that [nuclear energy] will ever be obtainable. It would mean that the atom would have to be shattered at will.”
— Albert Einstein, 1934.
“Anyone who expects a source of power from these atoms is talking moonshine.”
— Ernest Rutherford, 1933.
“There is no likelihood that man can ever tap the power of the atom… The glib supposition of utilizing atomic energy when our coal has run out is a completely unscientific utopian dream.”
— Robert Millikan, 1928.
These uncharacteristically myopic statements bring to mind one of Arthur C. Clarke’s famous laws of scientific research: “When a distinguished but elderly scientist states that something is possible, he is almost certainly right. When he states that something is impossible, he is very probably wrong.”
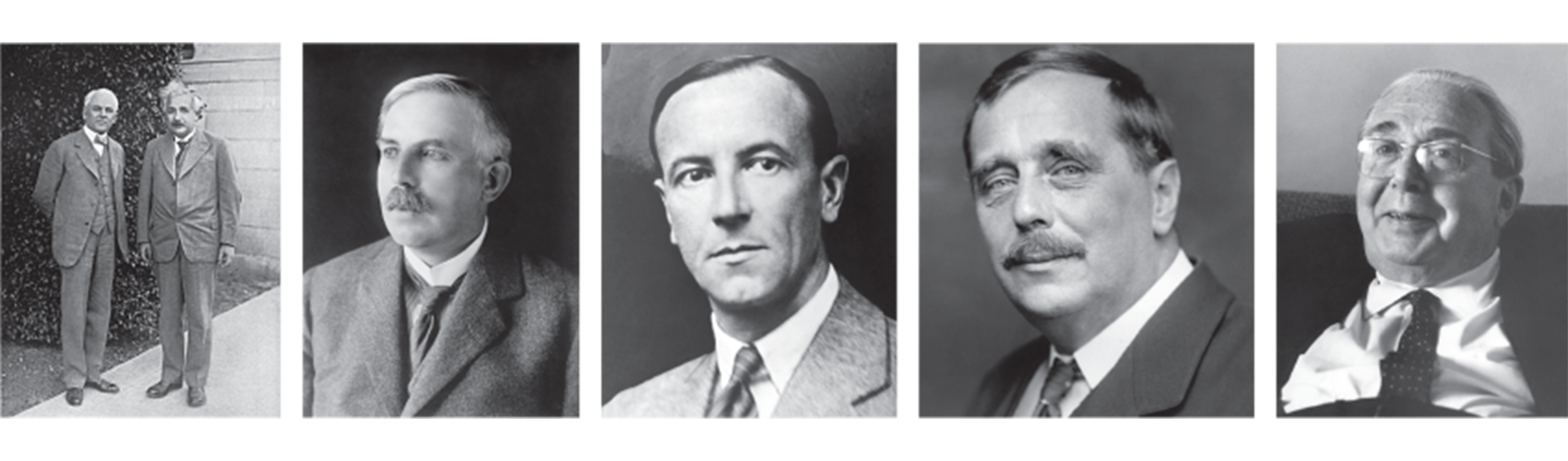
“All through the nineteenth and twentieth centuries the amount of energy that men were able to command was continually increasing. Applied to warfare that meant that the power to inflict a blow, the power to destroy, was continually increasing.”
— H.G. Wells, The World Set Free, 1914.
Life imitating art: Speculative fiction and fact
Although scientists don’t always manage to predict the future very well, speculative fiction writers often pick up the slack. In an impressive moment of prescience, H.G. Wells had coined the term “atomic bomb” in 1914 in his utopian novel The World Set Free—describing it as a hand grenade made from uranium which never stopped exploding. Wells was a keen follower of scientific developments of his era and unlike the scientists did not have to let technical realities get in the way of his imagination.
This idea proved to be influential. One of his biggest readers was Winston Churchill, who had credited Wells for coming up with the idea of using aeroplanes and tanks in combat ahead of World War I. In a 1924 article, Churchill ominously wrote, “Might a bomb no bigger than an orange be found to possess a secret power to destroy a whole block of buildings—nay to concentrate the force of a thousand tons of cordite and blast a township at a stroke?”
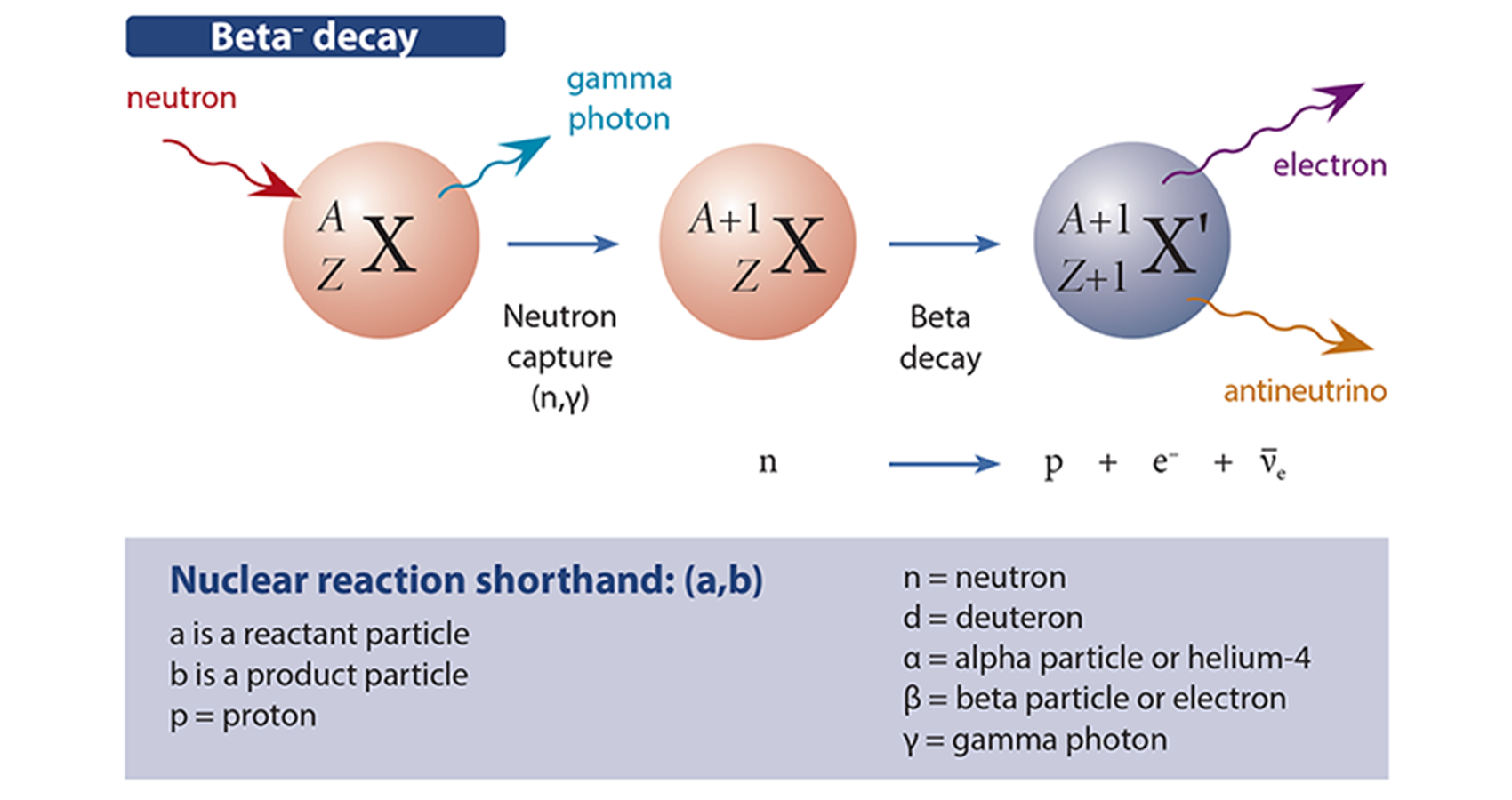
What changed to bring this idea from fiction to reality?
The discovery of the neutron by James Chadwick in 1932 in Rutherford’s laboratory opened the possibility of nuclear fission and firmly established the field of nuclear physics. In fact, Rutherford had long anticipated the existence of an uncharged particle of about the mass of a proton. The neutron was revealed to be a component of the atomic nucleus, where it is stable, but can also exist free for short periods (around 15 minutes).
This discovery became important because neutrons could be fired at and captured by the atomic nucleus, and transform its configuration via beta decay (note, a β– particle is an electron). This is an important process, proposed by Enrico Fermi in 1933, in which a neutron decays into a proton, electron, and an antineutrino (see figure above). The latter two are quickly lost, and therefore the nucleus increases in atomic number (Z). In effect, you put a neutron into an atomic nucleus and it turns into a proton—a useful trick. At the time, however, Fermi had difficulty publishing his theory, which deeply frustrated him. Nature rejected it on the grounds that it seemed crazy.
Five months prior to the announcement of the neutron, Harold Urey and co-authors published a paper describing naturally occurring “heavy hydrogen,” later termed deuterium, in which the hydrogen nucleus is one atomic mass unit heavier. It became apparent after Chadwick's discovery that this heavier isotope contained a neutron and a proton—a hydrogen nucleus normally just consists of a single proton.
During this revolutionary year, the Hungarian physicist Leo Szilard happened to read Wells’ The World Set Free, which appeared to him in a new light after these discoveries. Described as an eccentric and a “one-man idea factory,” Szilard suddenly came up with the technological mechanism of the atomic bomb in September 1933—the chain reaction—while watching the traffic lights turn green in London. He wrote, “It suddenly occurred to me that if we could find an element which is split by neutrons and which would emit two neutrons when it absorbed one neutron, such an element, if assembled in sufficiently large mass, could sustain a nuclear chain reaction.” He filed for a patent on it in 1934, which he later turned over to the British Admiralty in 1938. In his memoirs he wrote, “Knowing what it would mean—and I knew because I had read H.G. Wells—I did not want this patent to become public.”
The neutron alchemists
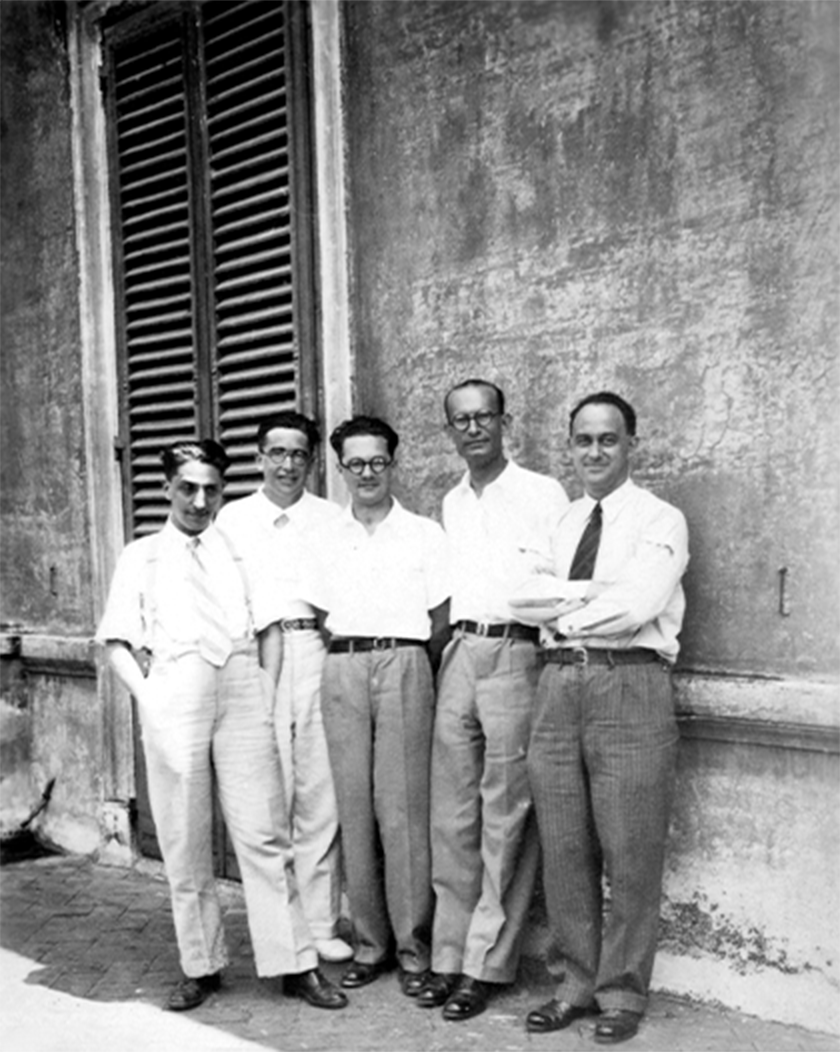
Enrico Fermi was a brilliant Italian physicist with wide-ranging interests, known primarily at the time for his work in theory. In 1934, his focus switched to experimental physics as he began his work using neutrons, emulating the methods of Irène and Frédéric Joliot-Curie who had induced radioactivity in elements by bombarding them with alpha particles. His proposal was that charge-neutral particles (neutrons) could penetrate the cationic nucleus better than positively charged alpha particles would. Not only was he wildly successful with this approach, but it opened the way to completely unanticipated developments.
Starting with the lightest elements systematically and working upwards with increasing atomic number, within months his group in Rome, known as the “I ragazzi di via Panisperna” (Via Panisperna Boys), had discovered that they could induce nuclear transformation in many of these compounds. This work led to the discovery of the principle of neutron moderation and the enhanced capture of slow neutrons, which they discovered by accident. The team noticed that if they bombarded an element while on a wooden table, it made that element more radioactive than when it was on a marble table. This remarkable observation was brought to Fermi, who was known jokingly within the group as “Il Papa”—the Pope—because of his infallibility. He speculated that the light hydrogen and carbon nuclei in the wood acted to slow down the neutrons, which increased the probability that they would spend more time in the atomic nucleus and damage it. Hence an increase in radioactivity. This was quickly confirmed using a block of paraffin between the neutron source and the target, and became the key to achieving transmutation of heavier elements.
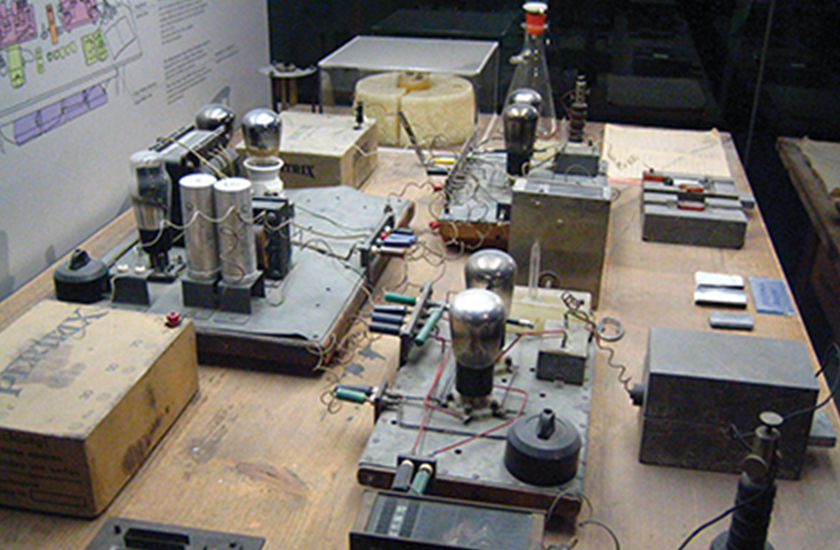
With light elements, they found that a neutron could knock out a proton (an n,p reaction) or an alpha particle (n,α). Heavier elements always gave neutron capture (n,γ). Following neutron capture, if the new nucleus was radioactive they observed that it always decayed by beta decay to form the next heaviest element.
Fermi and his team quickly made their way through the entire periodic table and arrived at the heaviest known element of the time, uranium. Because they observed beta emission following neutron irradiation, they reasonably believed that they had produced the next heaviest transuranic elements 93 and 94 but could not identify them definitively due to limitations of their radiochemistry techniques. Initially, Fermi was uncomfortable about their lack of evidence for these conjectured elements—an extraordinary claim—and refused to name them. Nevertheless, he published a paper entitled “Possible production of elements of atomic number higher than 92” and included them several years later in his 1938 Nobel talk, dubbing them ausonium and hesperium—Greek names for Italy.
The timing turned out to be unfortunate. Shortly after Fermi received the Nobel Prize “for his demonstrations of the existence of new radioactive elements produced by neutron irradiation,” Otto Hahn, Lise Meitner, Fritz Strassmann, and Otto Frisch upturned his results in Berlin and simultaneously described nuclear fission. They were investigating Fermi’s reaction with uranium and slow neutrons but identified the products not as transuranics but as lighter fission products—barium and krypton.

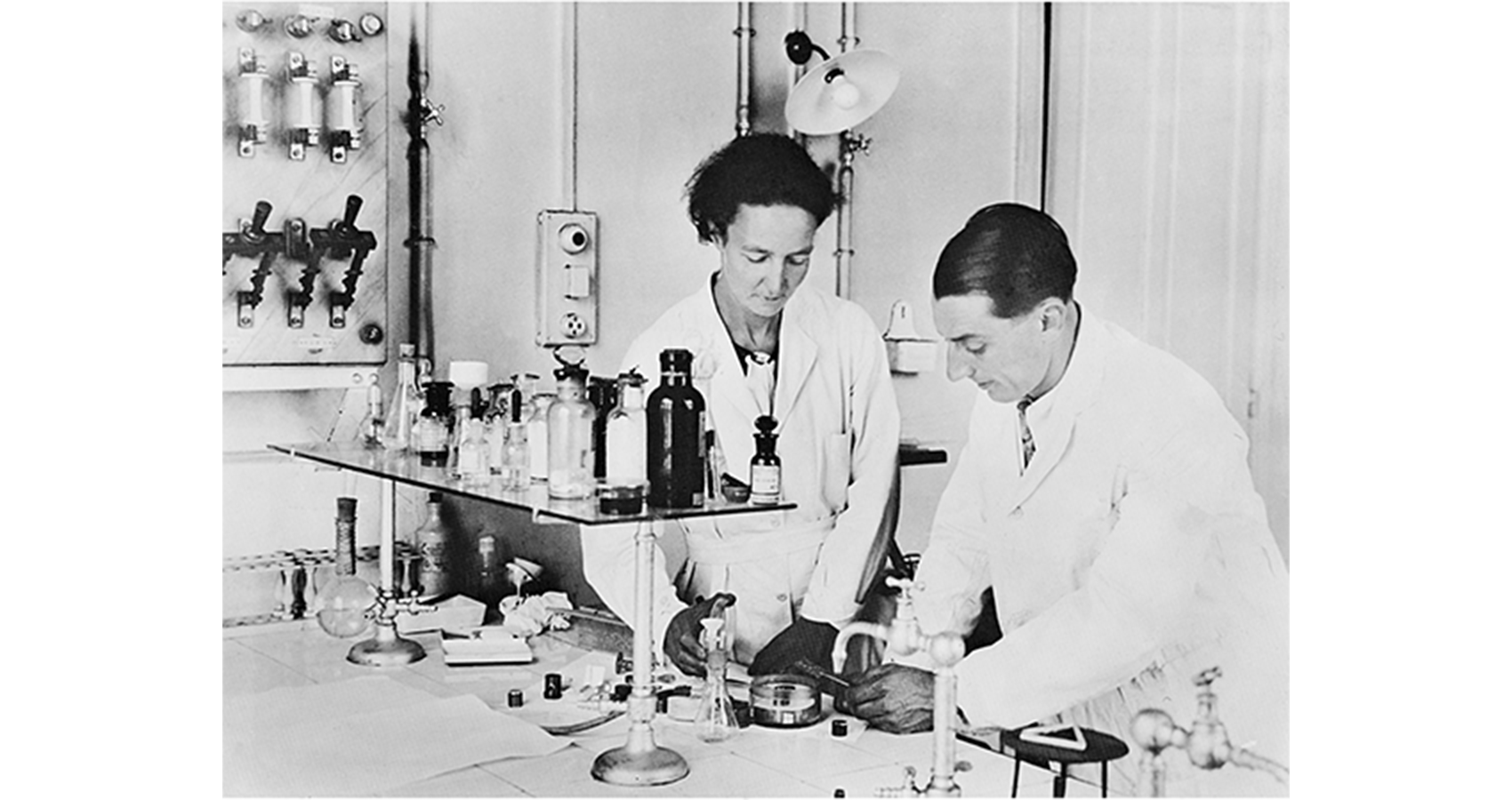
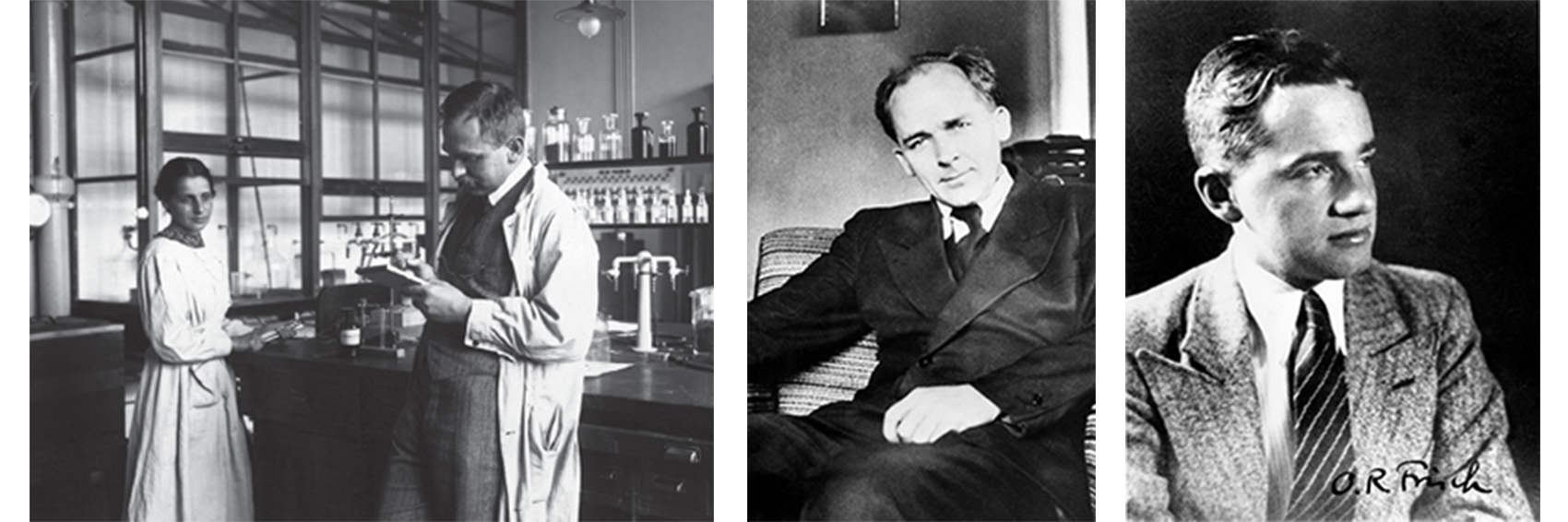
“As chemists we are obliged to accept the assignment of barium to the observed activity, but as nuclear chemists working very closely to the field of physics we cannot yet bring ourselves to take such a drastic step, which goes against all previous experience in nuclear physics. It could be, however, that a series of strange coincidences has misled us.”
— O. Hahn, F. Strassmann, Naturwissenschaften, 1939, 27, p11.
Fermi had originally disregarded the possibility of fission based on his calculations but had overlooked a component of binding energy. Furthermore, his team used lead shielding on their target elements, which had hidden the strong electromagnetic pulse emitted upon fission. He humbly added a corrective footnote to his written Nobel lecture when he sent it to the printer that winter.
This overturned long-held beliefs in physics and paved the way for plutonium. Many have wondered how history could have turned out differently if Fermi had successfully discovered fission in 1934. Perhaps Germany would have developed the nuclear bomb first, or maybe both sides would have reached this point at the same time, resulting in a different form of cold war. We can only speculate.
Experiment had leapt ahead of theory meanwhile and it was the turn of the theoreticians to catch up. The father of atomic theory, Niels Bohr, had an epiphany about the nuclear fission of uranium on a snowy walk across the Princeton campus in 1939. His brilliant insight was that the phenomenon was owing to uranium-235, a minor isotopic component of the natural ore. The liquid drop model, developed and published with John Wheeler in that year, also made it possible to predict that element 94-239 would also be fissile.
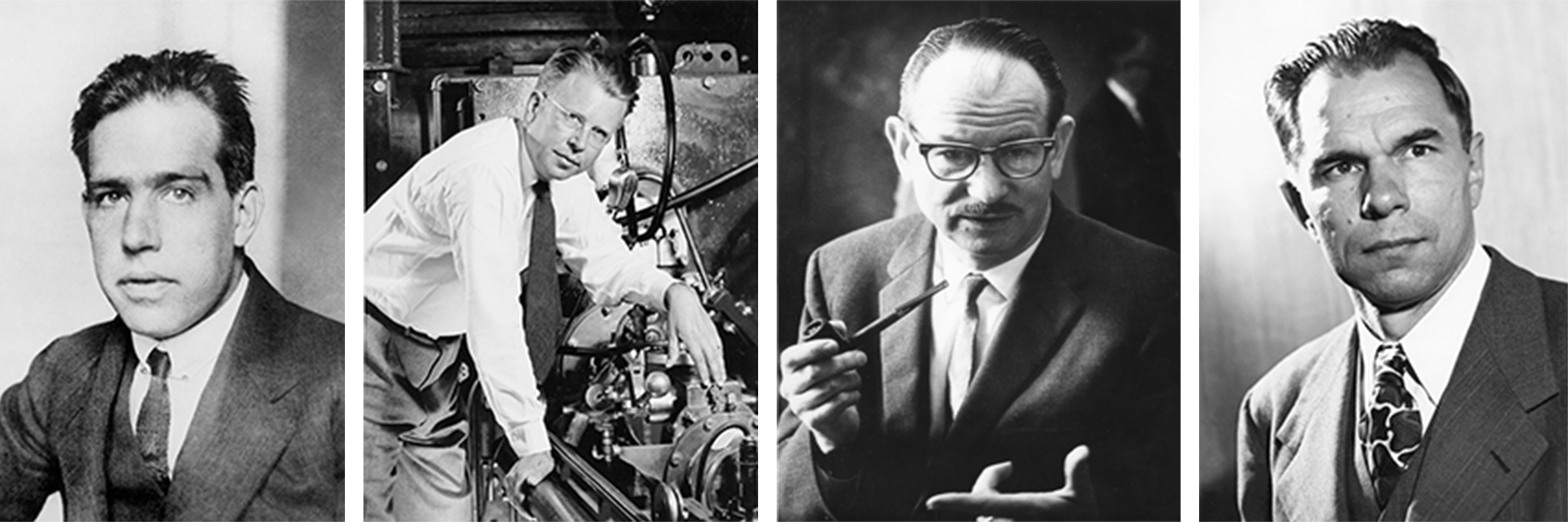
Neptunium and beyond
Scientists had not abandoned the pursuit of new transuranic elements using Fermi’s neutron methods. Although neutron absorption can lead to fission, as shown by the Berlin researchers, it can also result in neutron capture. Therefore, transmutation of uranium to elements 93 or 94 could still occur via addition of neutrons and beta decay if the source of neutrons was tuned for neutron capture.
Using a new, powerful cyclotron, a type of particle accelerator invented by Ernest O. Lawrence in 1929 at the University of California Berkeley, Edwin McMillan and Philip Abelson successfully characterized element 93 in 1940. They duly named it neptunium, following uranium. The cyclotron was used to slow the neutrons, which were then fired at a uranium-238 target, leaving a characteristic 2.3 day activity (half-life) which did not scatter away from the target like a typical fission product. This was identified as neptunium-239, the product of beta decay of uranium-239. Unfortunately, neptunium was too short-lived to be very useful, but the researchers suspected it was decaying into an even heavier element.
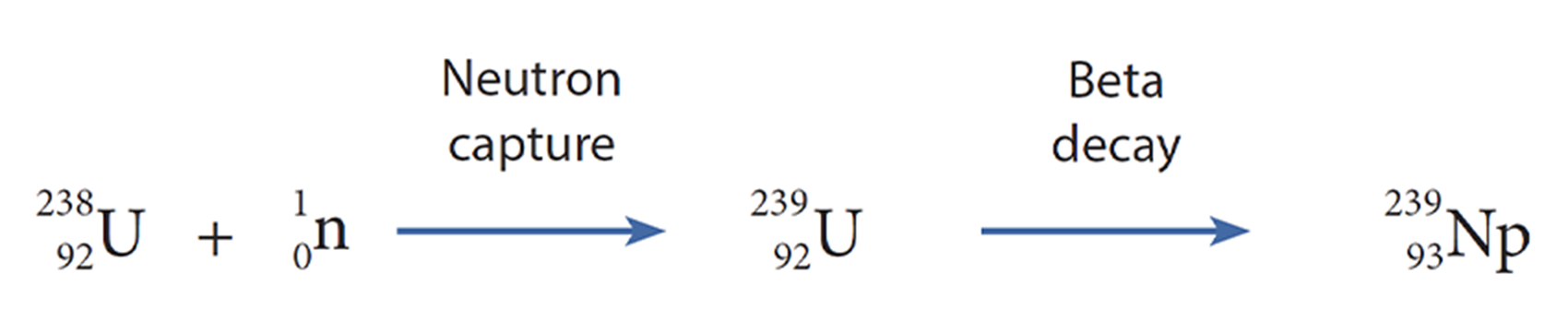
McMillan was called away to MIT in the fall of 1940 to develop radar as part of an anticipatory war effort, given the looming inevitability that the US would join World War II. At the time he had been looking for the daughter of neptunium’s beta decay, which calculations dictated should be element 94, and had been pursuing the bombardment of uranium with deuterons with a similar goal. A deuteron is a deuterium nucleus, which contains one proton and one neutron. Because it has a positive charge, they needed high bombardment energies (16 MeV) to overcome the Coulombic repulsion; this energy was so high that two neutrons were emitted. Using this (d,2n) pathway they calculated the lighter isotope neptunium-238 as product, which could lead to the hypothetical species 94-238. Shortly before he was called away, he had found tantalizing evidence indicating this new transuranic element.
One of McMillan’s colleagues, Glenn Seaborg, had been following this work closely. When McMillan moved to the east coast, Seaborg wrote a letter asking whether he could continue the cyclotron research—Ed replied saying he would be happy to turn the work over. Seaborg kept a close correspondence with McMillan on the work throughout. Seaborg’s team—including Joseph Kennedy and Arthur Wahl— made impressively quick progress. The first deuteron bombardment took place on December 14, 1940. Just ten weeks later, on the night of February 23, 1941, element 94 was unambiguously identified. The deuteron route had proved to be more tractable than the neutron pathway because it yielded the shorter-lived isotope 94-238, rather than 94-239. This less-stable isotope gave a stronger signal via alpha emission and was therefore easier to identify. The critical challenge they overcame was the ultramicrochemical separation on a microgram scale, which they solved with a novel chemical oxidation process developed with Berkeley chemist Wendell Latimer’s help. The discovery that plutonium fluoride was insoluble, and therefore separable, would later become an especially important secret a few years later under the Manhattan Project.
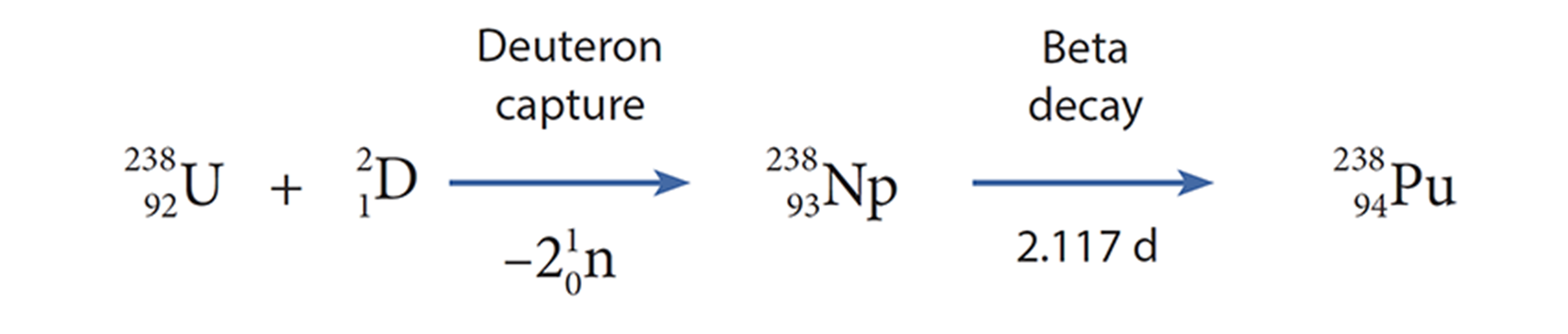
The team were obviously thrilled. Seaborg said “we felt like shouting our discovery from the rooftop,” but they knew that could not happen. When McMillan and Abelson had published the discovery of neptunium in 1940, the British government had lodged a protest, complaining that Americans were revealing potential military secrets. And element 94 could be far more impactful than 93.
They submitted the finding to the Uranium Committee, an organization that Roosevelt had established in 1939 to facilitate communication with scientists and determine the feasibility of a nuclear chain reaction. Seaborg then contacted Physical Review to place a temporary hold on the publication of their findings and went back to work. The revelation of their discovery to the world would eventually come in the most dramatic way imaginable.
The fission mission
Before they had even finished characterizing the new element, the researchers wanted to test it for fissionability—the key question to be answered. However, they required the heavier isotope, 94-239, instead of 94-238, which they had initially synthesized. To achieve this, they had to bombard their target with neutrons instead of deuterons, i.e., McMillan’s original (n,γ) method which they had still been exploring in parallel. The challenge they faced was isolating neptunium-239 as an intermediate compound. It was therefore necessary to scale up the experiment to 1.2 kg of uranyl nitrate to produce just 1 μg of neptunium-239, which would theoretically undergo beta decay to an isolable sample of just 0.5 μg of 94-239. This was a scale-up of a factor of over 200 from the original 6 g scale and put the researchers in far greater danger with exposure to fission product radiation.
Lawrence assigned Emilio Segrè, an Italian émigré and a former student of Fermi’s, to assist Seaborg in this effort due to his record of accomplishment in the field. In 1937, Segrè had identified a new element, technetium, as the first artificially synthesized chemical element from a radiated strip of molybdenum that Lawrence had sent him.
Seaborg recalls that their shielding methods were “jury-rigged and unsophisticated.” They put their liquid sample in a large lead bucket, which Seaborg and Segrè carried precariously across a street and up a two-story stairwell on a long pole using lead-lined gloves and goggles for separations chemistry conducted on an open-air balcony. After some challenging chemical workup, they left the sample of neptunium to decay for three weeks before they reached the moment of truth—a watershed moment that was significant not just for science but the whole world.
On March 28, 1941, after they had improved the purity and determined the sample was free of uranium-235, they put it back into the cyclotron for neutron bombardment. The results were obvious—the pulses registered on their meter showed that it was undoubtedly fissile. A few months later they designed an experiment that measured the all-important fission rate, and finally they had their answer: 94-239 was even more fissile than uranium-235, consistent with Bohr and Wheeler’s theory. Their initial value gave 1.7 times uranium-235, an overestimate compared with the modern value of 1.24, but the conclusion was the same.
By making a fissile material out of inert uranium-238, which constitutes 99% of natural uranium ore, they had found a far easier and potentially scalable route to making atomic bombs. The trick for scientists would be to find that route—a cyclotron could not be used for large-scale manufacture.
“As soon as such a machine [reactor] is in operation, the question of how to obtain explosive material, according to an idea of von Weizsäcker takes a new turn. In the transmutation of the uranium in the machine, a new substance comes into existence, element 94, which is very probably—just like 92U235—an explosive of equally unimaginable force. This substance is much easier to obtain from uranium than 92U235, however, since it can be separated from uranium by chemical means.”
— Werner Heisenberg, February 26, 1942, lecture to an audience that included high-ranking Nazi officials.
German progress
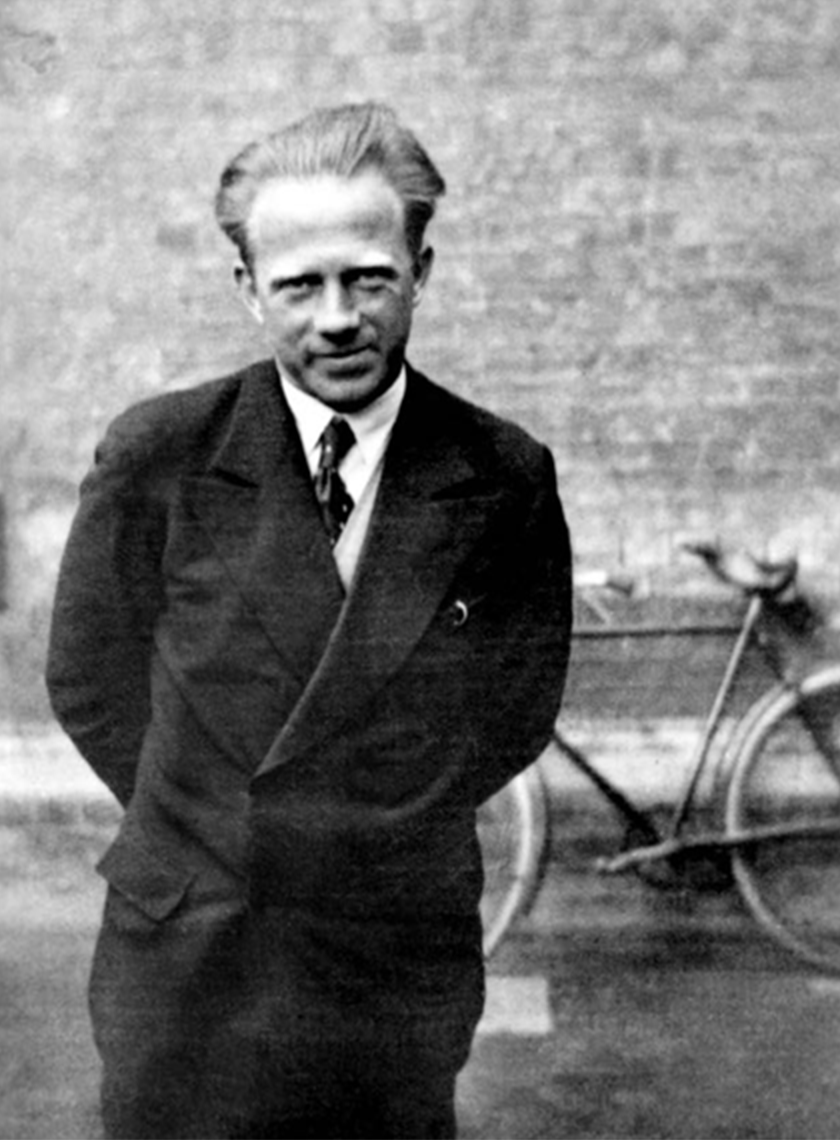
Courtesy: AIP Emilio Segrè Visual Archives.
One of the main driving forces that formed and fueled work under the Manhattan Project was the threat that the Germans would get there first. This was a very rational fear—nuclear fission had been discovered in Berlin after all. And central Europe was the epicenter of the recent atomic physics revolution with Germany producing some of the most famous scientists in this field, such as Max Born (Robert Oppenheimer’s graduate advisor), Werner Heisenberg, Hans Geiger, Max Planck, Albert Einstein, and Fritz London. Although some of these physicists had left Nazi Germany, many remained and were happy to collaborate with the Nazi party.
After the discovery of nuclear fission in 1939, the German War Office had established a program, the “Uranverein” (Uranium Club), to develop nuclear energy, which included developing explosives. In 1941, the German Secretary of State Ernst von Weizsäcker (father of the famous physicist Carl Friedrich) filed a highly speculative patent that included a description of element 94 as a nuclear explosive derived from uranium-238. Although the general ideas were correct, the patent completely lacked any of the essential technical details and the Germans do not appear to have followed up on this effort. Throughout this period, Kurt Starke and the discoverers of fission, Hahn and Strassmann, were working on transuranic elements. In 1942, they confirmed the discovery of neptunium but did not pursue element 94, although it is likely that they were aware that it should be fissile. This could have been because they knew about McMillan and Abelson’s lack of success in isolating it in their original 1940 paper on neptunium. Another major reason for their decision could have been that they lacked access to a sufficiently strong neutron source. Historians now view the lack of cyclotron technology as a major obstacle that prevented Axis forces from acquiring nuclear weapons technology before the Allies.
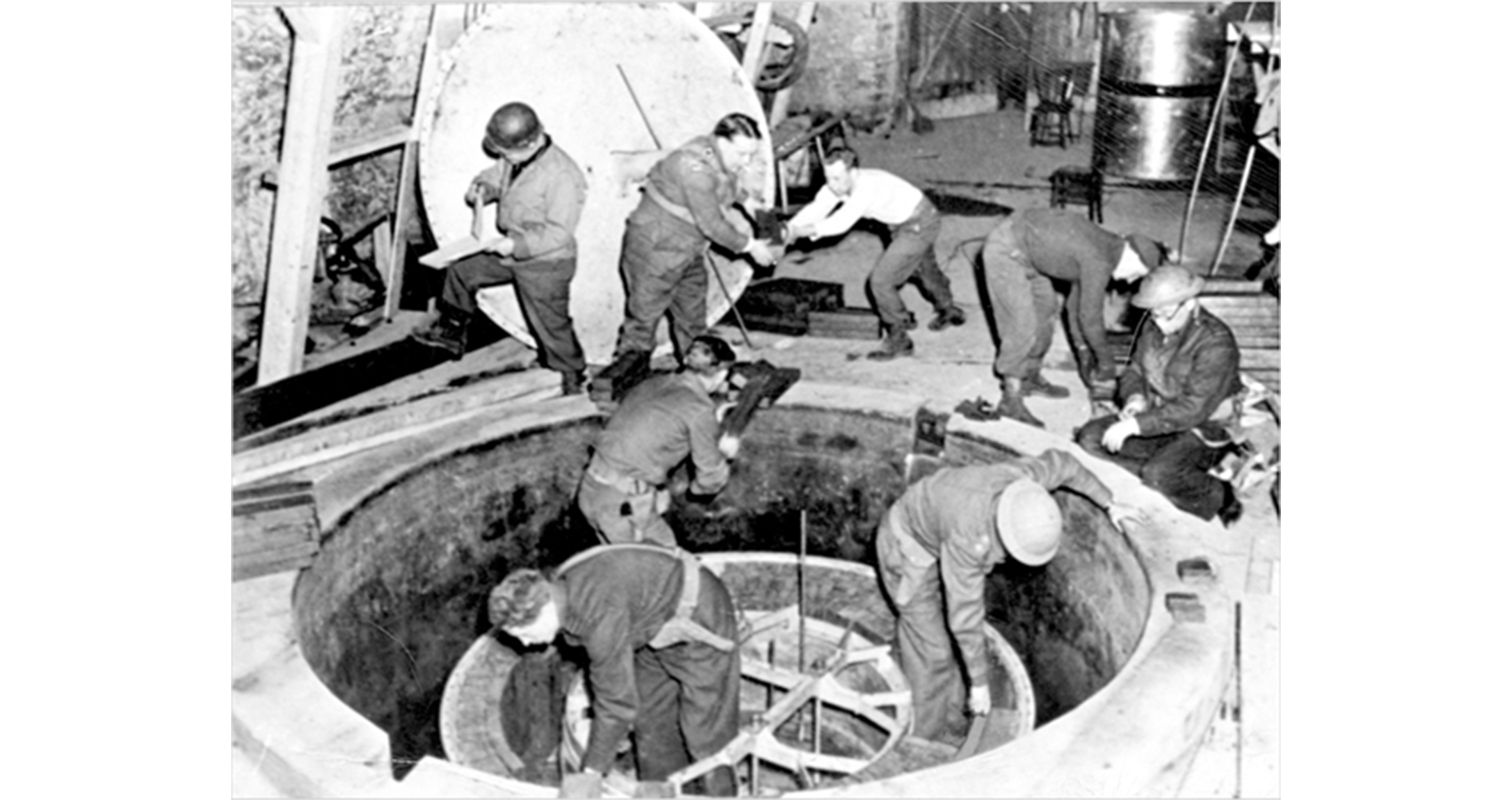
Secret baptism of 94, or “49”
In April, 1942, Seaborg joined Fermi at the Metallurgical Laboratory in the University of Chicago as part of the brand new, and of course extremely secret, Manhattan Project effort. In this same year, plutonium was unofficially named. Seaborg briefly considered dramatic names such as “extremium” and “ultimium”, as it was the heaviest element yet discovered, but bowed to the planetary tradition of naming the actinide (Pluto, which had only been known for a decade, was considered a planet at this time).
Ironically, shortly after it gained its name it was quickly given codenames to disguise its identity. Names such as “49” (a reversal of its atomic number) and “copper” were used, but sometimes proved to be confusing—for instance, when they had to use real copper it had to be referred to as “honest-to-God copper”!
1942 turned out to be another landmark year for nuclear physics. Fermi had moved to Columbia University in 1939 and there, by great fortune, met Szilard, who shared his idea for the nuclear chain reaction and persuaded Fermi to pursue it experimentally.
In one of the most famous experiments in scientific history, on December 2, 1942, Fermi and his team demonstrated a self-sustaining nuclear chain reaction using “a crude pile of black bricks and wooden timbers,” in his own words. The bricks were graphite neutron moderators—45,000 of them—used to slow the neutrons down, much like the paraffin wax they had used previously, and the fuel was a mixture of uranium metal and oxide in a carefully calculated heterogenous lattice arrangement (called a pile as the components were piled up). The experiment only ran for half an hour before its growing heat and radioactivity became dangerous, but it was enough.
The demonstration of a feasible nuclear reactor would have in more ordinary times heralded the beginning of a new age of nuclear power. However, given the race to an atomic bomb against the Germans, Fermi’s achievement had a more pressing application—a scalable production method for plutonium.
Micrograms to kilograms
Thanks to the work of Seaborg’s team, scientists knew something about the nuclear properties of plutonium-239—its half-life, fission rate, and nuclear crosssection, all estimated, however, with significant margins of error. But everything else remained unknown, and the design of a bomb would require the accurate determination of key properties such as density and compressibility, which would, in turn, determine the critical mass of the warhead components.
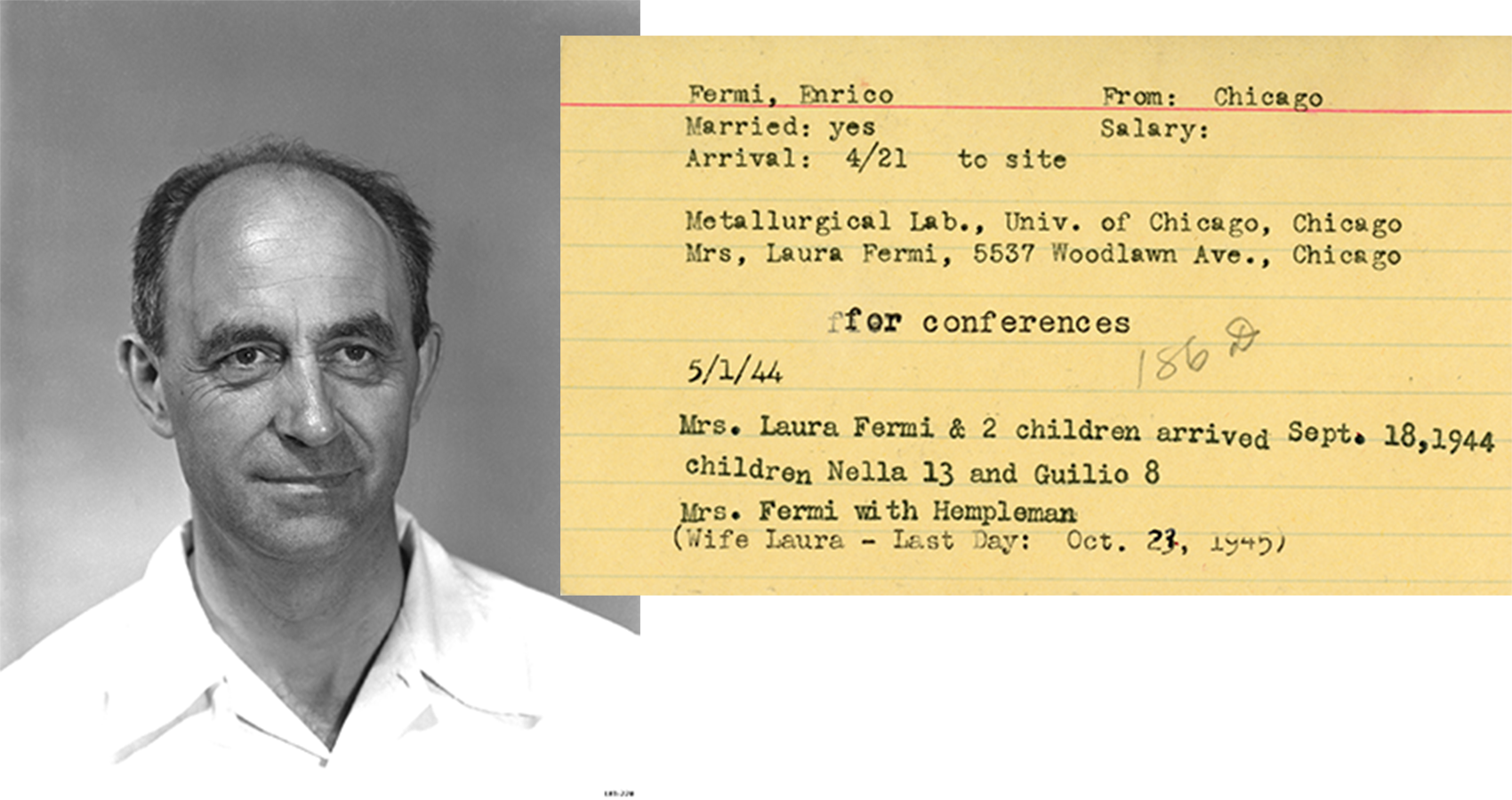
Little did they know the complexities that lay ahead for them—at the time, the assumption was that plutonium belonged to the transition metal series and therefore would have chemical properties in line with a heavy analog of osmium (see the historic periodic table on p31). Plutonium would, however, turn out to be the most complex element known to science.
There were two main technical challenges. First, how to scale up production to fabricate kilogram parts, a question largely of physics and engineering. Nevertheless, the difficulty of this undertaking was unprecedented—engineers had to design chemical processing plants costing hundreds of millions of dollars on the basis of a few experiments carried out with quantities of plutonium so minute as to be invisible.
“The Italian navigator has landed in the new world”
— Coded message conveying Fermi’s success.
The second challenge was how to isolate plutonium in pure samples, just a few parts per 10 million by weight for each of the lightest impurity elements, a problem which belonged to the domain of chemistry. Exceptionally pure plutonium metal was essential to avoid predetonation, or a “fizzle”, in which only a small proportion of the fissile material undergoes fission before the assembly is blown apart. Plutonium is a strong alpha emitter and when alpha particles collide with certain nuclei from impurities, neutrons are created, i.e., an (α,n) reaction.
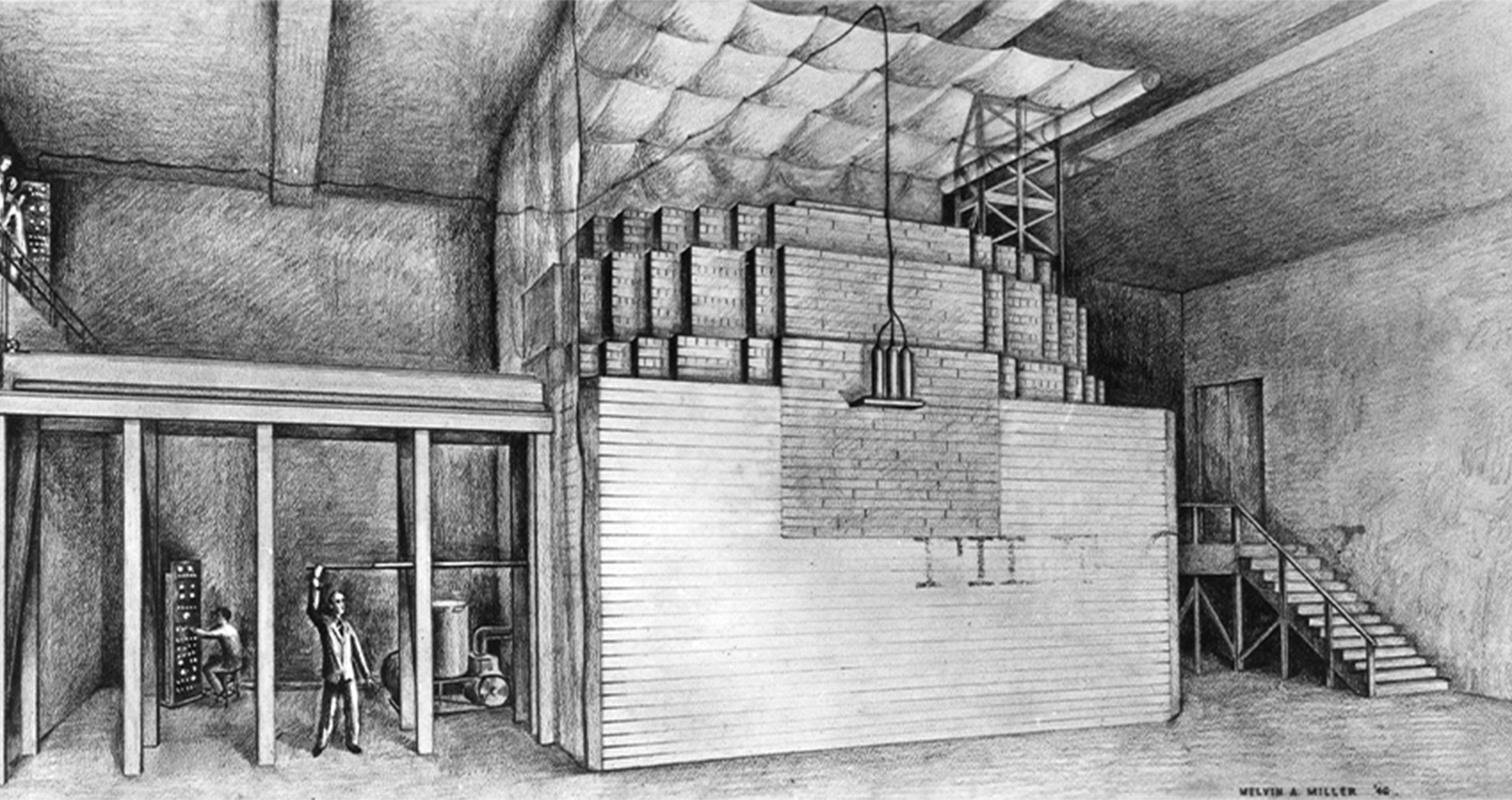
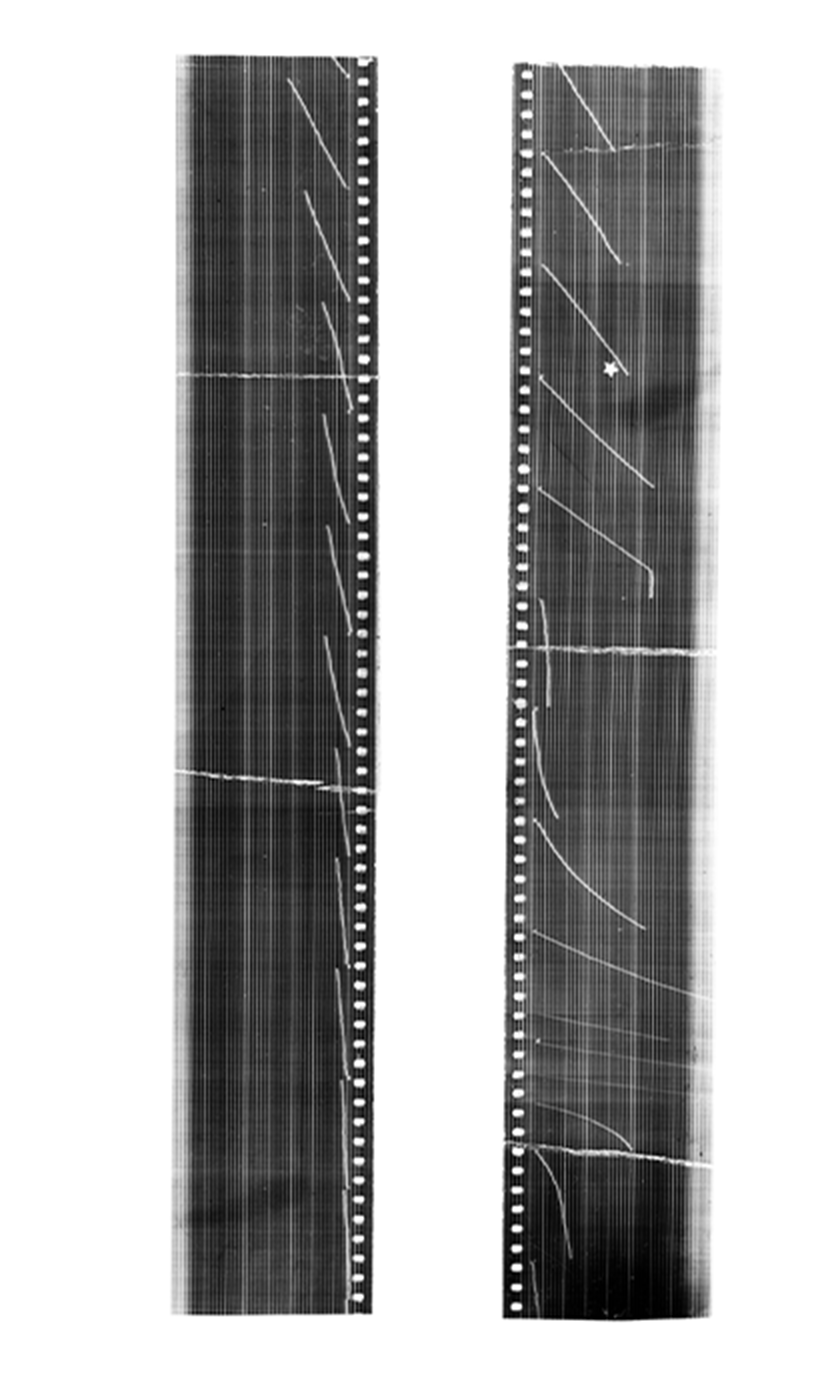
Before either of these issues could be addressed, they needed to scale up the quantities of plutonium available simply for research purposes. Fundamental research efforts were being hampered by access to even milligram quantities of the new element.
On August 18, 1942, they had their first big success. They were able to create a trace quantity of plutonium that was visible to the eye—a pink sample of the fluoride salt. Within weeks, on September 10, the first sample of PuO2 was weighed at 2.77 μg. This was no mean task. Burris Cunningham had re-invented the Salvioni balance, originally created in 1901 for measuring the limits of olfactory detection, using a quartz fiber just 0.06 mm in diameter and 12 cm in length. The weight of the sample was determined by measuring the amount of bending in the fiber. Some of his colleagues referred to this as weighing “invisible material with an invisible balance.”
This impossibly tiny sample was the first time a weighable sample of any type of product had been produced by a particle accelerator. It was part of a production batch of several hundred micrograms derived from hundreds of pounds of uranium using the Washington University cyclotron in St. Louis, MO, which was by then being used to bombard uranium ore 24 hours a day, 365 days a year. The lanthanum fluoride oxidation-reduction extraction procedure was used for the separation of plutonium from fission products and gave yields upwards of 90%. With this knowledge they determined plutonium's atomic weight.
Finding the density of plutonium was important—the critical mass is highly dependent on density (e.g., for a spherical solid, critical mass varies with density ρ as 1/ρ2). The variations in measurements that they observed instead created a great deal of confusion. Even as the amounts of plutonium grew to gram-scale buttons by 1944 (see p32 for images), and with it the capacity of repeated experimentation, the measured density still varied from 13 to 22 g/cm3. For reference, lead has a density of 11 g/cm3—plutonium is very dense! These inconsistencies were the first clue to the complexity of the element, which exhibits profound and non-classical allotropism. The researchers did not fully understand this at the time and had to eliminate other factors, such as impurities, from their inquiries.
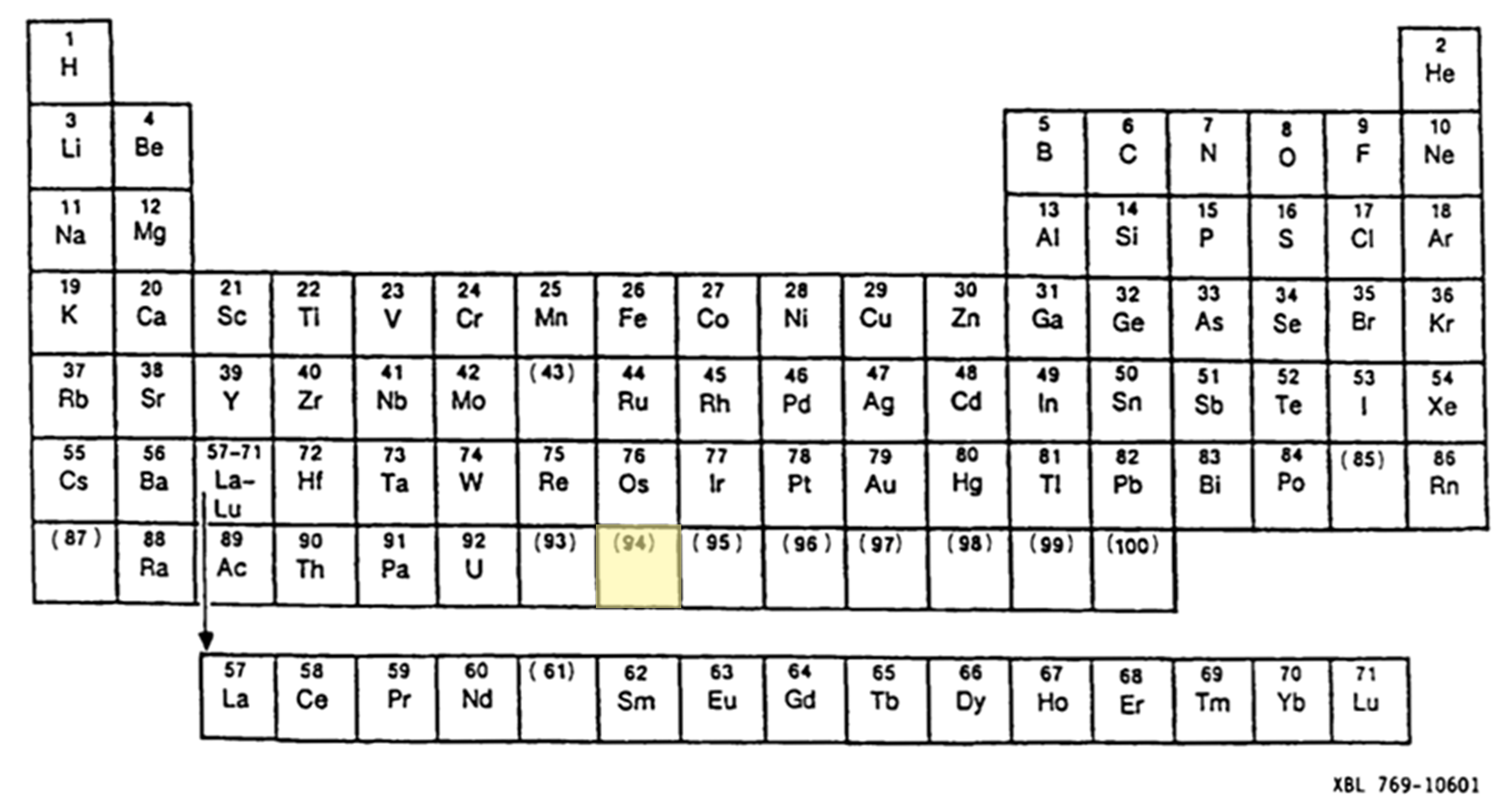
Extraction process
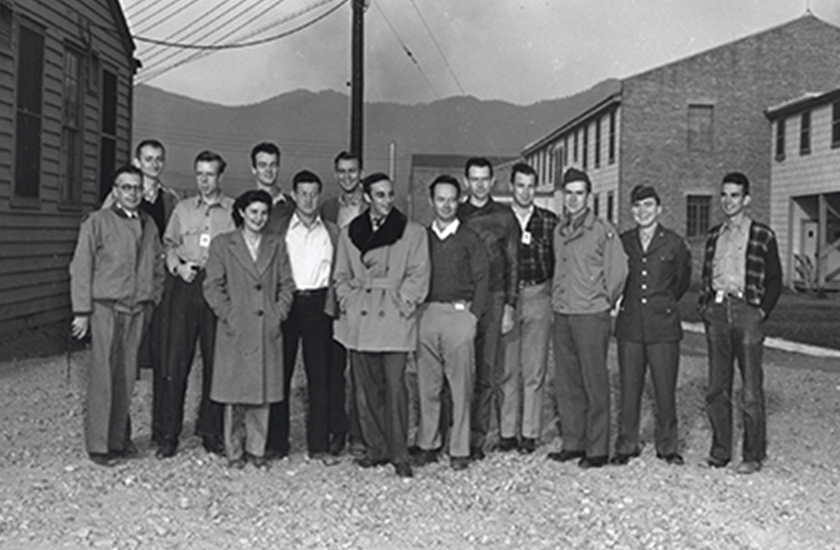
Seaborg and his team had originally extracted plutonium using an oxidationreduction protocol after establishing that the new element had higher and lower oxidation states that showed different chemical properties. This was performed in the aqueous phase with the objective to achieve separation via precipitation of a solid. On the tiny scales, this was only possible if they coprecipitated the product using a neutral “carrier”—a method previously pioneered by McMillan and Abelson for the extraction of neptunium using cerium fluoride. In the case of plutonium, lanthanum fluoride was found to effectively “carry” the lower oxidation state of plutonium, which was obtained as the fluoride salt.
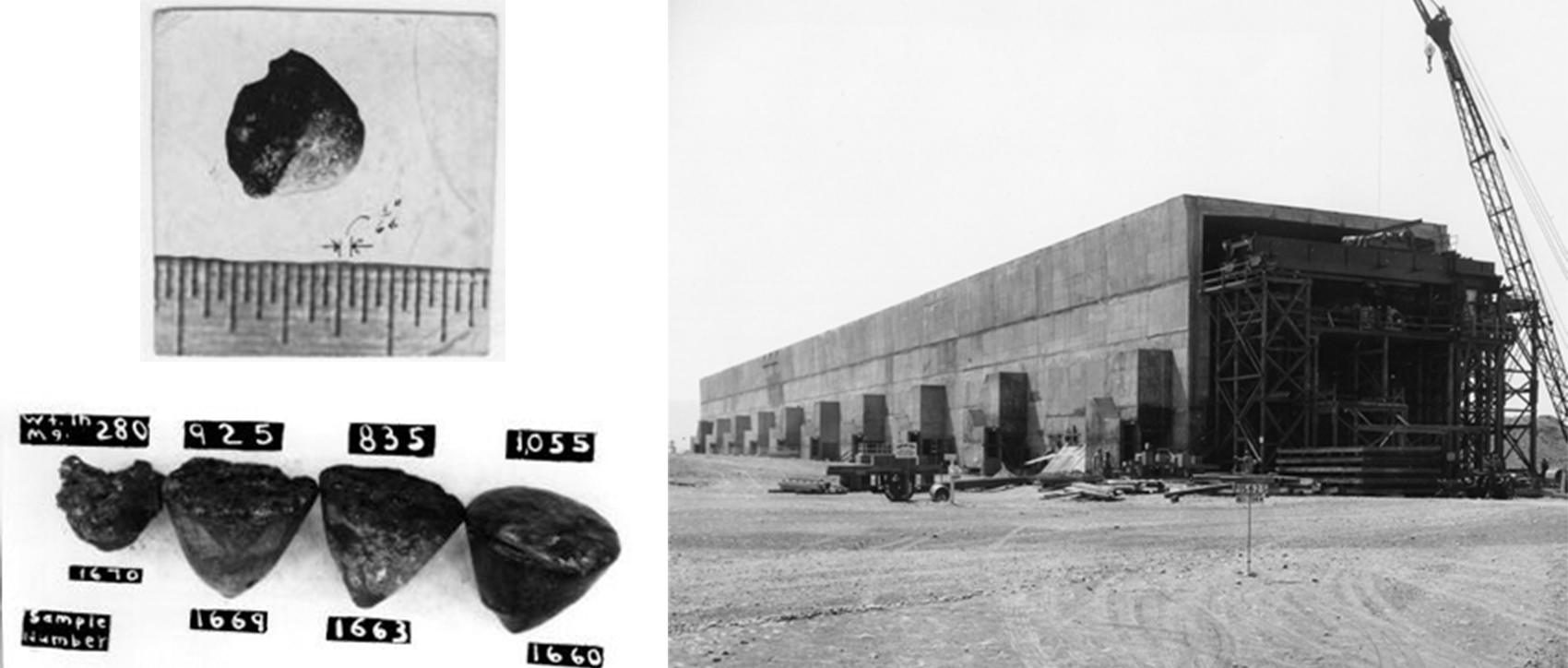
Right: The Hanford T Plant: the world's first large-scale plutonium separation facility, nicknamed the Queen Mary after the ocean liner. Operations began in December, 1944.
Along with its sister U Plant, these were scaled-up versions of the semiworks at Oak Ridge, each containing separation and concentration buildings in addition to ventilation and waste storage areas. The buildings were 800 feet long, 65 feet wide, and 80 feet high, containing 40 massive process pools. T Plant was designed to process about a half-pound of plutonium metal from one ton of irradiated uranium each day.
The processes were controlled remotely behind thick concrete walls and leaded glass. This included the world’s first CCTV system and early robotics.
In early 1943, the low and high oxidation states were identified as +4 and +6, which parallel the most common states of uranium. Conversely, unlike in uranium, the high +6 state in plutonium proved to be far more easily reduced. This allowed the use of mild reducing agents which reacted only with plutonium and not uranium, facilitating separation. Two more states, +3 and +5, were found in 1944, which became useful for postwar extraction technologies, described later.
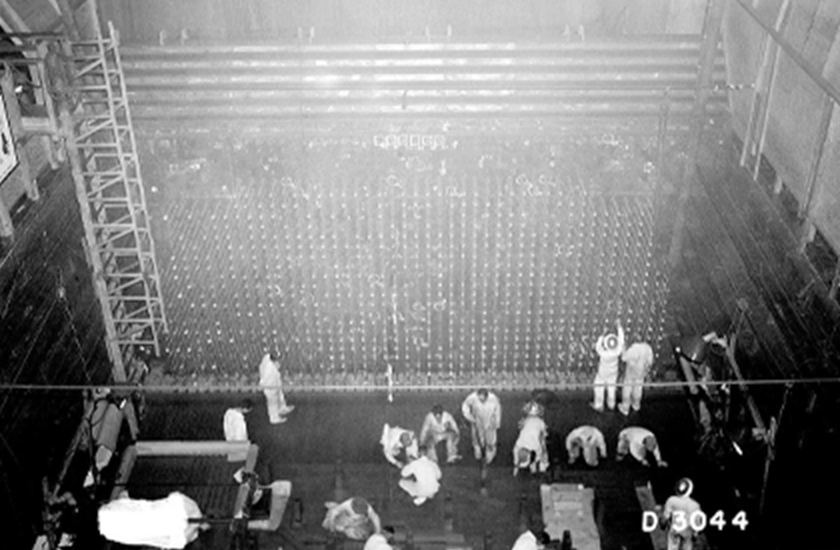
The reactor was graphite-moderated and water cooled, consisting of a 28 by 36-foot, 1,200-ton graphite pile, penetrated through its entire length horizontally by 2,004 aluminum process tubes containing uranium fuel slugs. Cooling water from the nearby Columbia River was pumped through the aluminum tubes around the uranium slugs. The design was based on the CP-1 prototype and X-10 pilot reactor in Oak Ridge.
Courtesy: Digital Photo Archive, Department of Energy, AIP Emilio Segrè Visual Archives.
The lanthanum fluoride process was used for all the cyclotron-produced plutonium up until 1943. Although very effective, it was also very corrosive, readily producing hydrofluoric acid upon hydrolysis. Therefore, a new process was designed for the anticipated scale-up of plutonium production. Screening a variety of phosphates, Stanley Thompson discovered in late 1942, with some surprise, that bismuth phosphate carried plutonium with 98% yield. The bismuth phosphate oxidation-reduction process, a variation on the original extraction method which gave plutonium peroxide as the product Pu(O2)2, was quickly adopted by the industrial separations plants which were being built for the Manhattan Project effort. Lanthanum fluoride was still used in smaller amounts for the penultimate “crossover” step to remove lanthanide impurities.
From accelerators to reactors
The first reactor to produce plutonium-239 was the X-10 Graphite Reactor in Oak Ridge, TN, which was based on Fermi’s prototype and began operations in 1943. In April, 1944, the first sample was available for testing. Worryingly, Segrè and his group determined that reactor-bred plutonium had a higher concentration of the hitherto-unknown isotope plutonium-240 than cyclotron-produced plutonium. This was an unfortunate blow for the bomb project—the rate of spontaneous fission was too high in this material for their gun design.
At this point, Oppenheimer became quite distraught at the prospects of success and seriously considered resigning as director of the project. General Groves persuaded him to stay and the project quickly pivoted. The impurities in the reactorproduced plutonium meant that they had to innovate a new implosion design instead of the more basic gun design that was being developed for uranium-235.
There were two important consequences of this. First, the limits of metal purity could be significantly relaxed. More problematically, they still needed to be able to cast it into a spherical or hemispherical form and precisely machine plutonium components. Coarse surfaces can introduce interfacial hydrodynamic instabilities and jetting under explosively driven high strain rates.
The allotrope problem
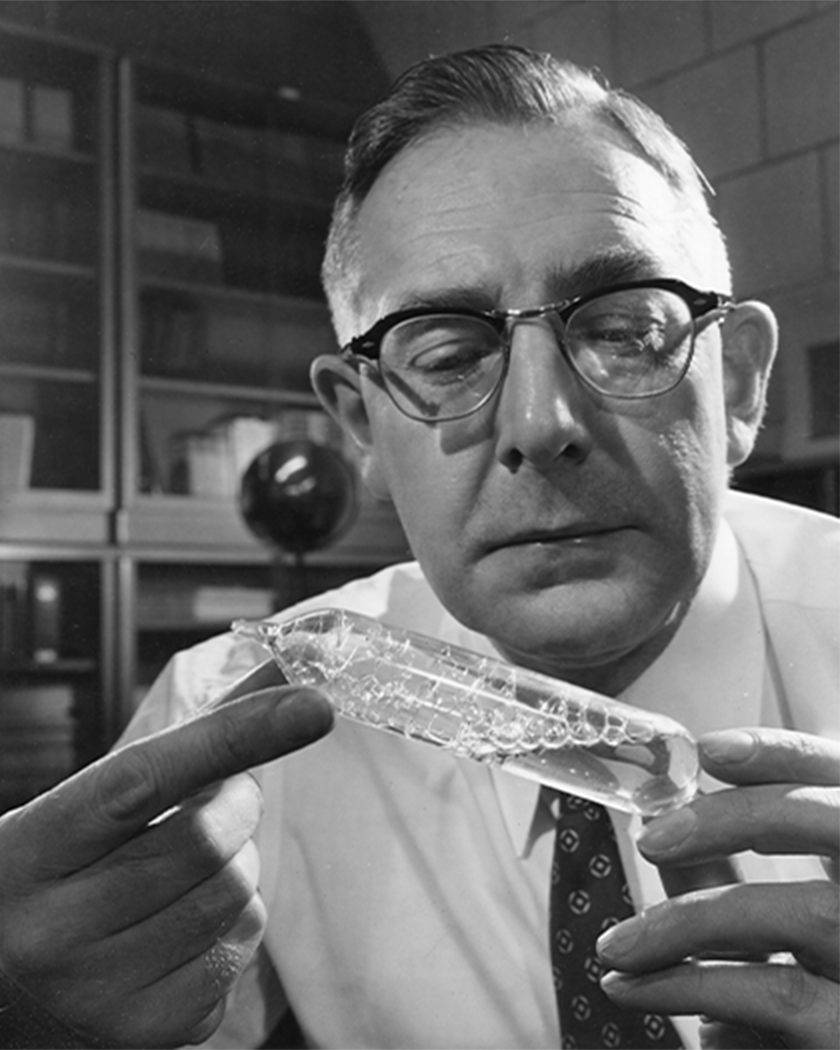
A major increase in plutonium production occurred in September 1944, with the startup of the Hanford B Reactor in Washington state, followed by the D and F reactors in the following months and achieving 90% yield by February 1945. The first delivery of Hanford plutonium was received by Los Alamos on February 2, 1945. During this period from early 1944 to mid-1945, enabled by increased access to the metal, Manhattan Project scientists made enormous progress in understanding the chemistry and metallurgy of plutonium. In particular, they found five of the six ambient-pressure phases, which helped them to solve the component machining problem in astonishing speed.
At room temperature, plutonium adopts the brittle monoclinic alpha phase, which is extremely difficult to manipulate—more mineral than metal. As fortunewould have it, the face-centered cubic delta phase had completely different properties and could be precisely machined like aluminum; unfortunately, it is only accessible at higher temperatures (317–453°C under ambient pressure). They achieved some success by machining at these temperatures, but as the design became more complex, these methods became unreliable, particularly as the metal changes by an astounding 20% in volume between the two phases.
Fortunately, there was a solution.
Cyril Smith, experienced in brass alloys, began an alloy survey program in 1944 to investigate the room temperature stabilization of the plutonium delta phase using an additive. There was little theory that could predict this type of metallurgy and therefore they cast a broad net with much trial and error. Aluminum was the first success, and work proceeded to cast hemispheres using this Pu-Al alloy. Sadly, after several months it became apparent that the neutron flux from (α,n) reaction with aluminum was too great a risk to be tolerated—at most it could be used at 0.5-atomicpercent (at.%), which wasn’t enough.
“At approximately noon on July 15, 1945, at MacDonald’s Ranch near Alamogordo in New Mexico, I put the proper amount of gold foil between the two hemispheres of plutonium. My fingers were the last to touch those portentous bits of warm metal. The feeling remains with me to this day, thirty-six years later.”
— Cyril Smith recalling his last-minute fix to prevent jetting of the Trinity device.
In the spring of 1945, with just weeks to go before the scheduled Trinity test, they chose gallium as a replacement due to its position as the heavy congener in the periodic row below aluminum. This additive was used in a 3-at.% quantity (0.8-weight-percent). They did not even have time to test the new alloy for stability—it could have conceivably reverted to its thermodynamically stable alpha form—and Smith was forced to go on gut instinct that it would work.
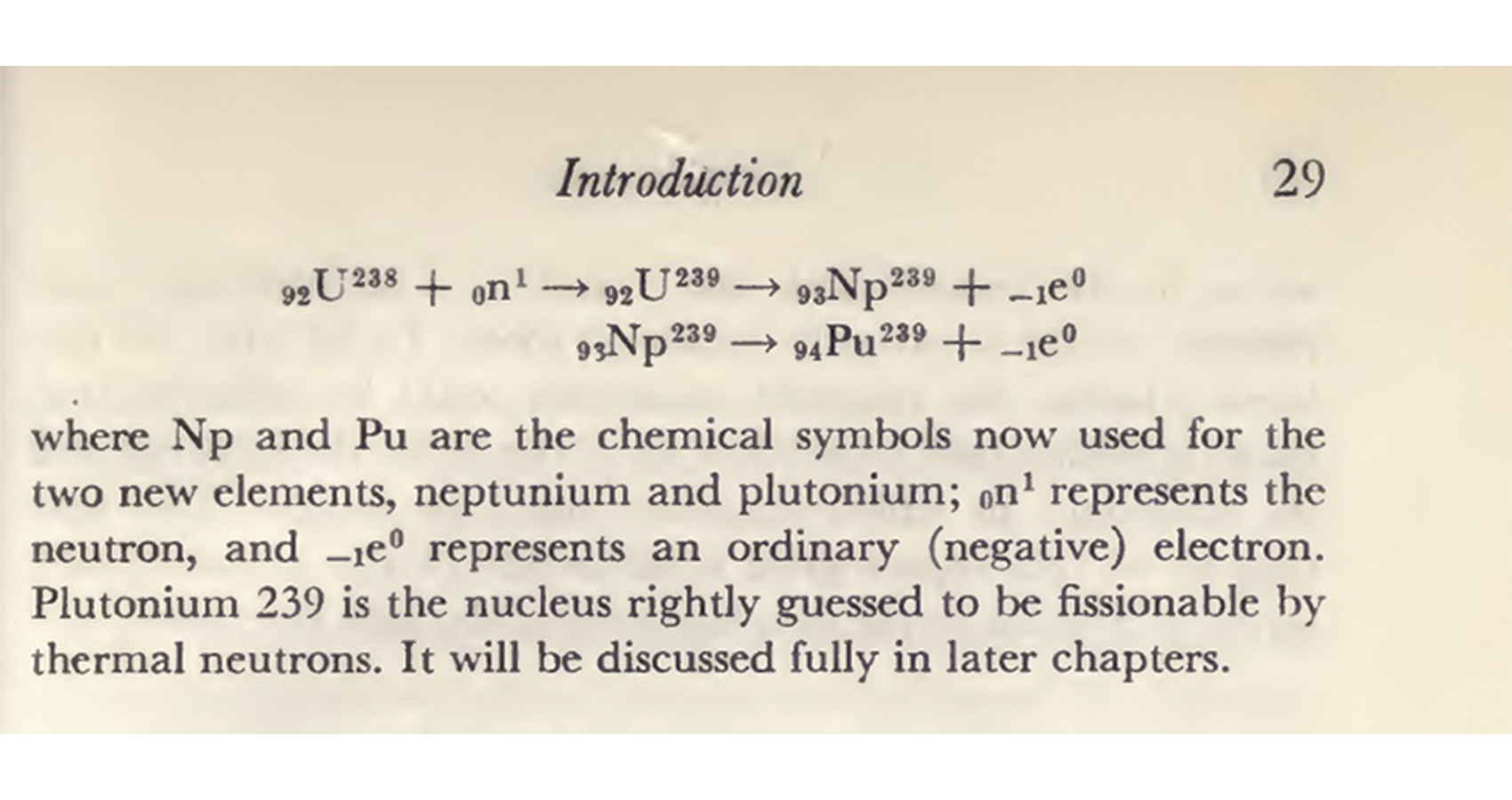
Plutonium announced to the world
On May 7, 1945, shortly before the Trinity test, Germany unconditionally surrendered to the Allies in Reims, France, ending World War II and the Third Reich. Seaborg describes this moment when “the United States no longer had to fear the enemy’s obtaining the bomb first.” In his book Adventures in the Atomic Age, he continued, “it was apparent that we could win the war without the bomb… For many Manhattan project scientists [this event] changed the moral tenor of the bomb research.”
Seaborg, Szilard, and others formalized their objections in the Franck Report and urged that “the use of nuclear bombs in this war be considered as a problem of long-range national policy rather than of military expediency.” The report anticipated the international proliferation of atomic weaponry, later dubbed “mutual assured destruction,” and warned against the policy of secrecy. They recommended that the power of the bomb be demonstrated on an uninhabited island rather than be used on Japan.
It is unlikely that Harry Truman saw this report, and instead kept the US and its new plutonium bomb on its original trajectory. On August 6, 1945, the uranium bomb Little Boy was dropped on Hiroshima, Japan, and just three days later the plutonium weapon Fat Man was unleashed on Nagasaki, with shocking levels of destruction. The Japanese surrendered on August 15.
Truman had been president for just four months, taking over from Roosevelt after his death on April 12, 1945. This was also the length of time he even knew of the existence of the Manhattan Project—Roosevelt had never seen fit to share this information with his next-in-command. Apparently surprised by the swiftness of the second bombing, Truman immediately issued an order to halt further use of atomic bombs without his express permission, revoking nuclear power from the military.
Only after the announcement of these first atomic bombs did the world learn of the existence of plutonium. On August 12, 1945, it was made known to the public by the Manhattan Project's Smyth Report.
What ifs
The story of the discovery of plutonium is full of surprises and serendipity, which carry with them enormous repercussions in geopolitical and civilizational history. We can wonder what if Fermi had discovered fission in Italy in 1934, when Mussolini was in power? Both Segrè and his colleague Edoardo Amaldi have suggested that fission could have been discovered as early as 1935. Or what if Fermi hadn’t met and collaborated with Szilard in 1939? What if plutonium did not lend itself to large-scale manufacture? Or what if the properties of the element were such that the delta phase could not be stabilized? How would Roosevelt have used the nuclear bomb if he had lived through his fourth term as president? And what kind of outcome would the Franck report have led to, if followed? And of course, the worry on the minds of all Manhattan Project scientists—what if the Germans had discovered plutonium first?
All these moments and decisions could have easily yielded a different outcome to World War II, something perhaps akin to Philip K. Dick’s counterfactual past in The Man in the High Castle. Nevertheless, this is how the cards unfolded in our history, which almost reads like science fiction in of itself.
Post-war
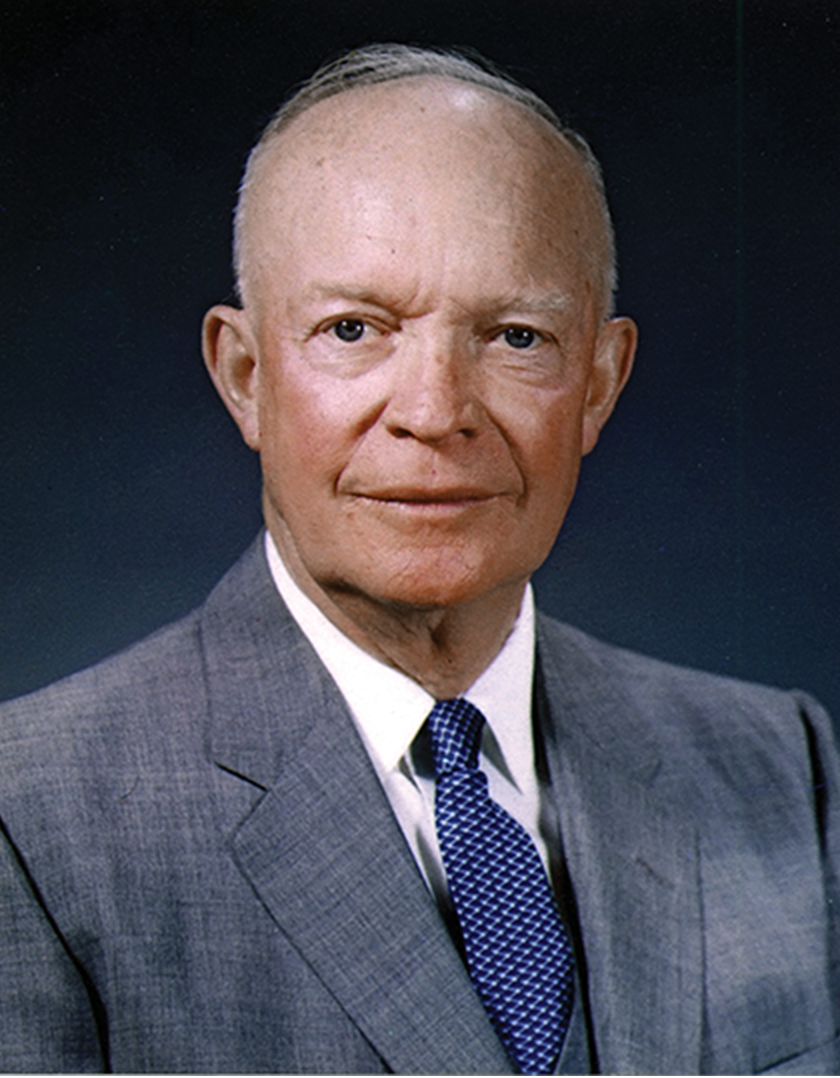
When President Eisenhower launched the Atoms for Peace program in 1953, much of the scientific and engineering research on plutonium became unclassified, except for details relating to weapons and certain manufacturing processes, which remain secret to this day. This was followed by the Geneva Conference on the Peaceful Uses of Atomic Energy in 1955, which resulted in the dissemination of information on separation processes for plutonium and its use in reactors.
“I feel impelled to speak today in a language that in a sense is new—one which I, who have spent so much of my life in the military profession, would have preferred never to use. That new language is the language of atomic warfare.” — US President Dwight D. Eisenhower, “Atoms for Peace” speech to the UN General Assembly in New York City, December 8, 1953.
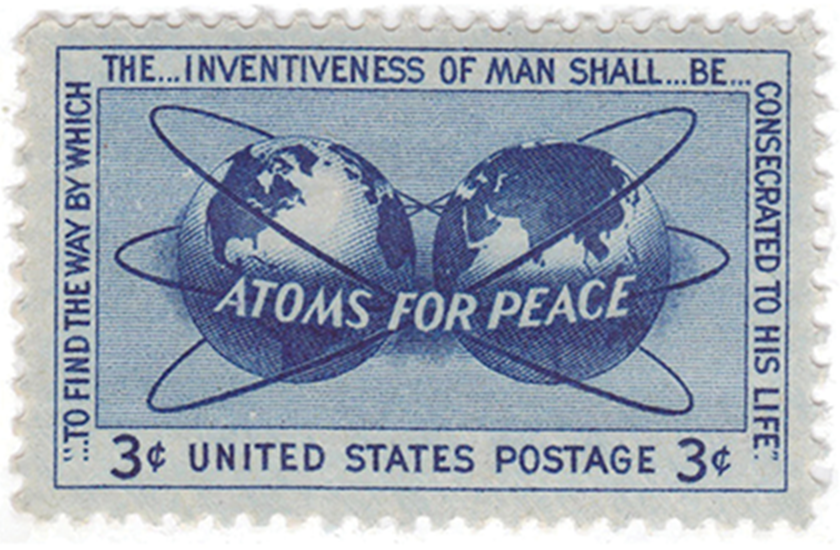
This opened the door to the first conference on plutonium science, The International Conference on Plutonium, held in Chicago, Illinois, in 1957. The Russians started discussing their results at the 1958 UN Peace Uses Conferences and at the subsequent international plutonium conferences in 1960, 1965, 1970 and 1975. The Actinides conference series began in 1981, in Pacific Grove, California, and in 1997, Los Alamos National Laboratory (LANL) established the Plutonium Futures biennial conference. The conference aims to enhance the dialogue among scientists and engineers on the fundamental properties of plutonium and on their technological consequences. The eleventh meeting occurs later this year, September 26–29, 2022, in Avignon, France.
In 1967, the American Nuclear Society published the Plutonium Handbook, edited by metallurgist O.J. Wick, collating all of the information available at the time and focusing on the peaceful uses of the element. It quickly became an indispensable resource for anyone working in the field. Fifty years later and a much-needed second edition was completed in 2019, edited by David Clark, David Geeson, and Robert Hanrahan, a huge collaborative effort weighing in at 3,500 pages.
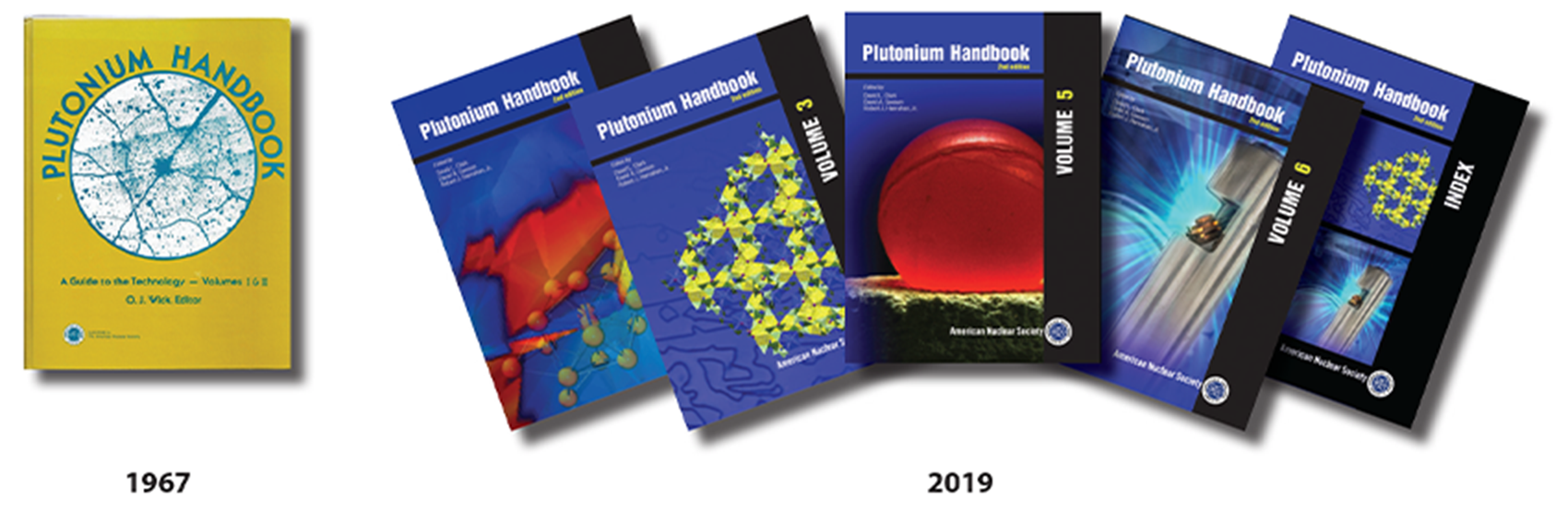
PUREX
Industrial production of plutonium scaled up considerably during the Cold War. It was revolutionized in 1956 by the switch at Hanford from the bismuth phosphate process, which was encumbered by its batch processing technique, to the PUREX (Plutonium Uranium Reduction EXtraction) method due to the much improved waste-to-product ratio (see D.L. Clark, “Chemistry challenges for the Manhattan Project and beyond”, Actinide Research Quarterly, Fourth Quarter, 2019, p20). We are still storing this high-level waste to this day—a major challenge to today’s chemists and engineers, and a significant obstacle to the widespread deployment of carbonneutral nuclear power.
The PUREX method was originally devised during the Manhattan Project by Herbert Anderson and Larned Asprey in 1947. It uses organic/aqueous separation, a reducing agent, and tributylphosphate as a ligand, which solubilizes certain plutonium and uranium species into the organic phase and separates them from fission products. Whereas the bismuth phosphate route relies on a +6/+4 redox process, the PUREX method reduces +4 to a +3 state. This low valent +3 species is not soluble in organic solvents—a key characteristic which allows phase separation. Once again, plutonium’s rich redox chemistry and ease of conversion between oxidation states, ostensibly a complicating factor, has been exploited to advantage.
Plutonium in the nuclear fuel cycle: Mixed-oxide (MOX) fuel
As part of the nuclear fission process, a proportion of uranium fuel converts to plutonium within reactors from neutron capture. After about 3–6 years in a reactor, when it is past its useful life and removed, the fuel contains approximately 0.8% uranium-235 and 1% plutonium, depending on the type used. To close the cycle, this spent oxide fuel can be reprocessed to isolate the plutonium oxide component. Workers then mill this together with uranium-238 oxide to created mixed-oxide (MOX) fuel. A single cycle of MOX fuel use increases the energy gained from the original uranium fuel by around 12%.
In 1963, the first MOX element was used in a Belgian light water reactor (LWR). Over 750 tons of MOX fuel have been used in LWRs, providing almost 5% of the new nuclear fuel today. In theory, this type of fuel can be used over and over again, but this has not yet been realized in practice.
The plutonium component can also be sourced from reprocessing fissile legacy materials in the stockpile, allowing for a productive use of decommissioned weapons via programs such as The Advanced Recovery and Integrated Extraction System (ARIES) at LANL. ARIES is a pit disassembly and conversion process that removes gallium and other impurities, and has to date converted over one metric ton of plutonium (see Actinide Research Quarterly, First Quarter 2008).
In the last decade, Russia has developed a new reprocessing system, REMIX, that does not separate the uranium and plutonium components in the spent nuclear fuel. The REMIX fuel can be repeatedly recycled and reprocessed up to five times, meaning that a reactor could run for 60 years on essentially the same batch of fuel.
Fast reactors
We obtain plutonium from uranium-fueled nuclear reactors, but it can also be used as fuel itself in fast reactors. These utilize fast neutrons, carrying energies above 1 MeV or greater, and can use either enriched uranium or plutonium-239. This concept was first demonstrated in 1946 in Los Alamos with the experimental prototype Clementine, which operated with the fission of plutonium-239. This was followed by EBR-I & EBR-II, LAMPRE-1, and Fermi-1 in the 1950s–60s. There are many such reactors in operation today, including prototypes designed for space travel, and most use MOX or uranium fuel—unalloyed plutonium has poor fuel characteristics from an engineering perspective.
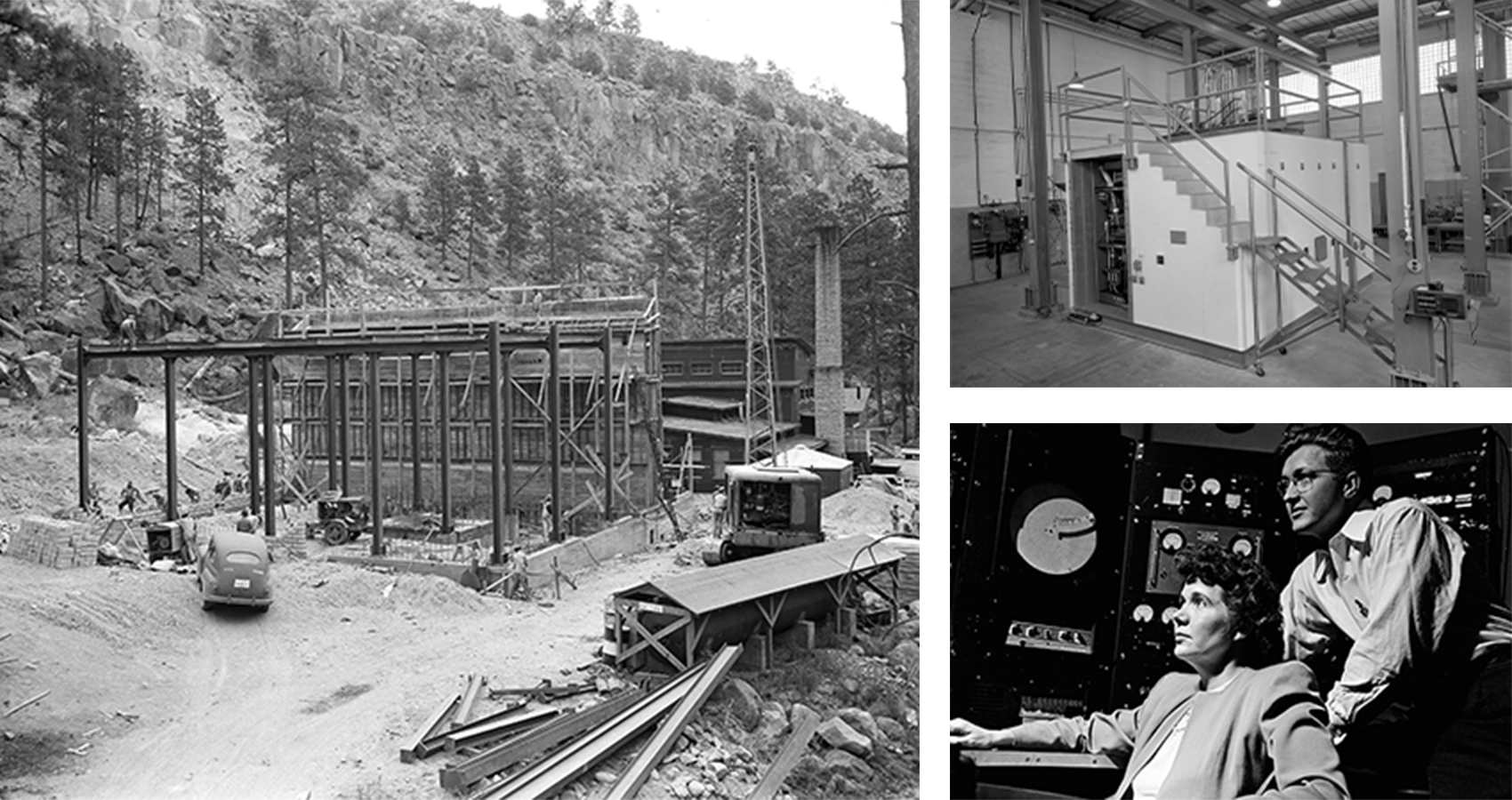
It also investigated the feasibility of civilian breeder reactors and measured neutron cross-sections of various materials. Husband and wife team Jane and David Hall (bottom right) were co-group leaders on the project.
Radioisotope thermoelectric generators (RTGs)
Plutonium-238 has proved to be the most useful of all known isotopes (including non-actinide) in radioisotope thermoelectric generator (RTG) applications. Exploiting the Seebeck effect, an RTG is a type of battery that produces electricity from the heat of radioactive decay. These batteries have specialized uses in unmanned applications in which long-lived, low-wattage, and lightweight power sources are required. Plutonium-238, used in the PuO2 form, is particularly suited for this due to its half-life of 87.7 years, reasonable power density of 0.57 W/g, and very low gamma and neutron radiation levels.
Engineers have used plutonium-based RTGs in satellites, beginning with Transit IV-A in 1961, and various forms of spacecraft, including NASA’s research probes Voyager 1 (1977), Voyager 2 (1977), Ulysses (1990), and Cassini (1997), and the Mars rovers Curiosity (2011; see Actinide Research Quarterly, First Quarter 2013) and Perseverance (2020). They also powered the Apollo Lunar Surface Experiments Package (ALSEP) in some of the Apollo missions (1969–1972). It is arguable that plutonium was essential for these lunar operations. After six decades of use, NASA has flown more than 25 missions carrying a plutonium-powered system. Future uses include the Dragonfly rotorcraft lander mission, which will explore Titan in 2034 (see illustration on p46). Due to the demand from NASA, the US restarted plutonium-238 production in 2013, which had ceased in 1988 with the end of the Cold War.
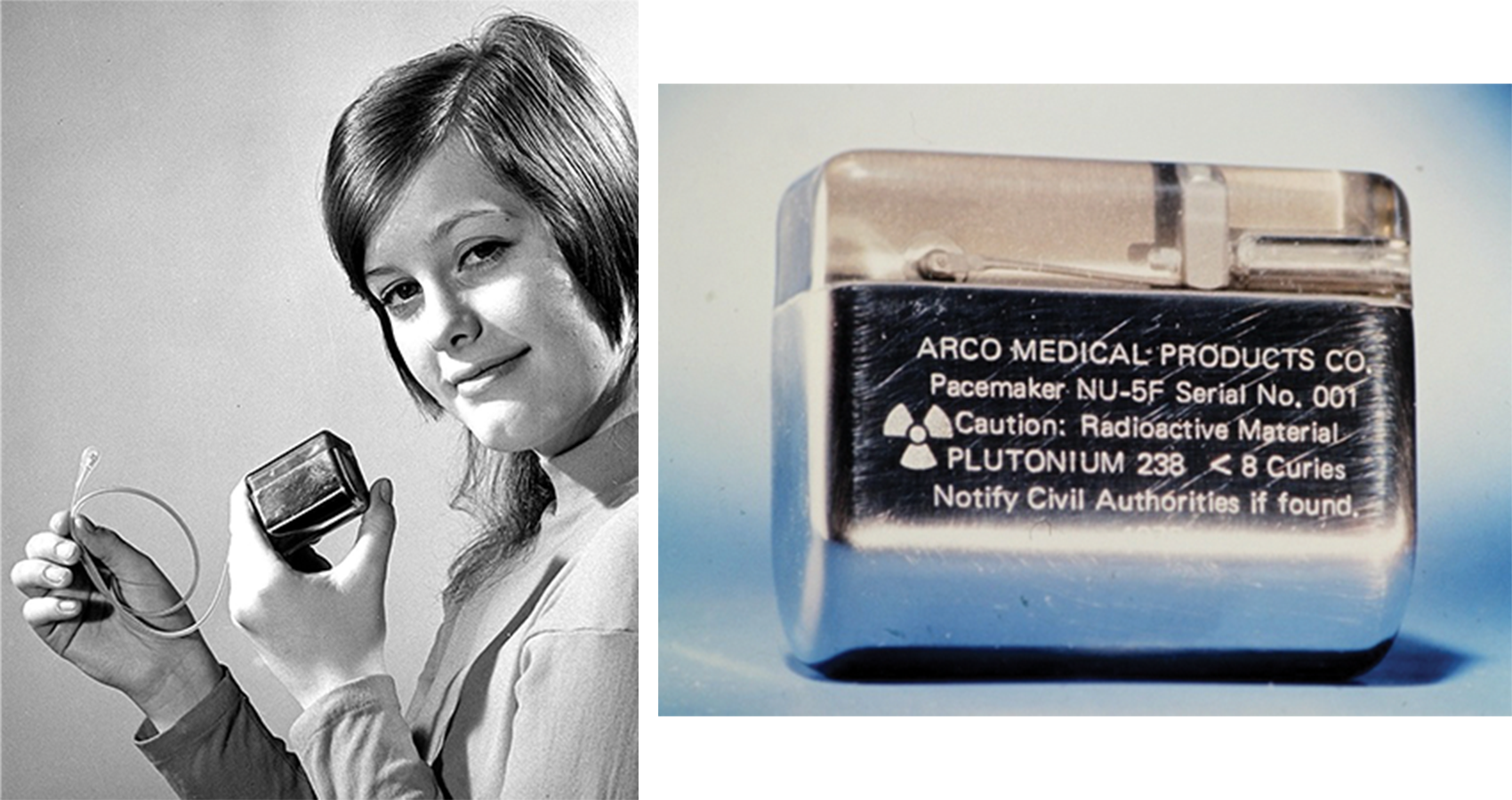
Right: The RTG battery in the historic ARCO Medical device (model NU-5F pacemaker) used a miniscule 8 Ci slug of metallic plutonium-238. This generated around 300 μW of power. Developed in the US by Numec Corporation under a contract from the US Atomic Energy Commission, it sold for $3,200 in 1974. Courtesy: ARCO Medical Products Co.
This type of plutonium RTG was also used in pacemakers, albeit in a very limited trial beginning in 1970 and ending in 1977. The dangers of radiation and the cost were the two main drawbacks, but the longevity of the battery—a true lifetime guarantee—remains undisputed. In 2007, Reuters reported that a plutonium-powered pacemaker implanted in 1973 was “still going strong after 34 years and may have saved money over the long run.” Nevertheless, lithium batteries have proven to be the superior choice for this technology and plutonium pacemakers remain a curious historical footnote.
“Plutonium is so unusual as to approach the unbelievable. Under some conditions it can be nearly as hard and brittle as glass; under others, as soft as plastic or lead. It will burn and crumble quickly to powder when heated in air, or slowly disintegrate when kept at room temperature. It undergoes no less than five phase transitions between room temperature and its melting point. Strangely enough, in two of its phases, plutonium actually contracts as it is being heated. It has no less than four oxidation states. It is unique among all of the chemical elements. And it is fiendishly toxic, even in small amounts.”
— G.T. Seaborg, “The first weighing of plutonium,” 1968.
Properties
Plutonium sits at an unusual spot in the periodic table. It is part of the actinide series, a sub-section of the f-block that is traditionally illustrated as an outcast, a floating island below the main rows of elements. As we traverse this period, the elements gain electrons in their 5f open shell, which also change in character and become more contracted. These 5f orbitals can be slippery to pin down, however, and are a major source of plutonium’s complexity.
Chemists often classify two idealized types of metals: simple ions, simplified as localized electrostatic charges (e.g., alkali metals), and covalent ions, which exhibit delocalized behavior such as covalent bonding (e.g., transition metals). The 4f orbitals of the lanthanides are so spatially contracted as can be considered to play little role in bonding interactions. Thus, lanthanides behave in terms of simple ions. This contrasts with the transition metals, meanwhile, whose d-orbitals are extended and play an active role in covalent bonding. The actinides bridge these two definitions, with early elements (e.g., uranium) behaving like transition metals because they possess more diffuse 5f orbitals and the later elements behaving like lanthanides due to the actinide contraction. Importantly, plutonium sits at the very equilibrium point where these orbitals move from a delocalized to a partially localized domain. This means that the specific properties of the metal are often dictated by the vagaries of quantum mechanics, leading to exotic states that are highly sensitive to external influences.
A large piece of plutonium feels warm to the touch because of the energy given off by 5 MeV from each alpha decay (plutonium-238 produces roughly 5.5 W/g); larger pieces can produce enough heat to boil water. Radioactive decay leaves behind structural defects and drives thermodynamics with local heating, creating uranium and helium atoms as impurities. Because of the helium buildup, storage containers need to be able to withstand pressure. Some but not all of the structural defects can be annealed out with temperature and time.
Plutonium predominantly emits alpha particles—a type of radiation that is easily stopped with a short range—and also neutrons, beta particles, and gamma rays. The alpha radiation makes it a serious internal hazard, made worse by its immobility in the body where it can remain for decades—as much as 80% of any amount absorbed will remain 50 years later. Just a few micrograms distributed through the lungs, liver, or bones can statistically increase the likelihood of cancer. This has contributed to its reputation as one of the most toxic substances known— the Department of Energy’s limit of occupational concentration in air is about a million times lower than for lead. Nonetheless, there are organic toxins that are many factors more deadly—plutonium absorption is mainly dangerous in the long term. Consequently, this aspect of plutonium can be managed with careful monitoring.
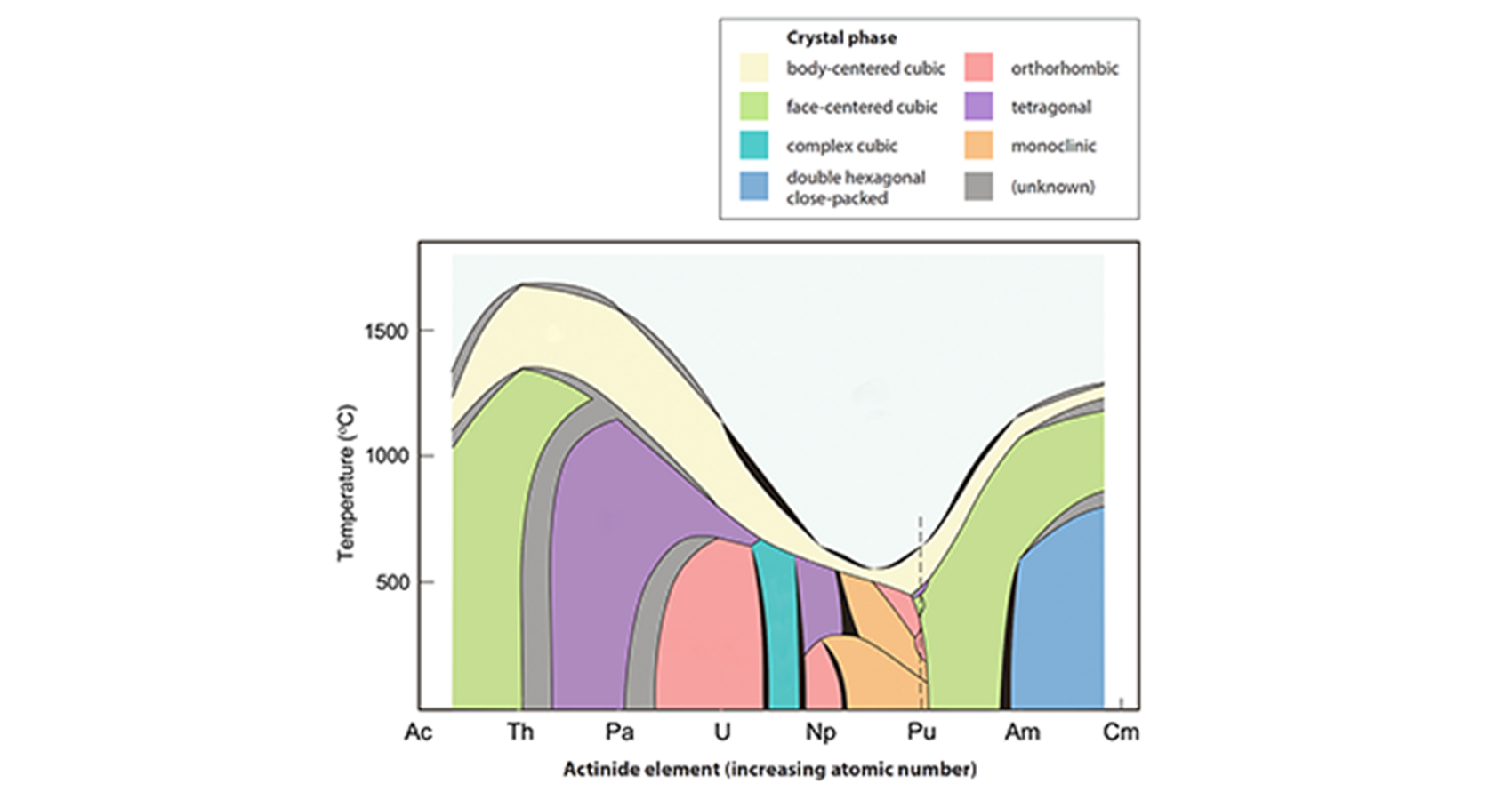
Adapted from “Magnetism or Bonding: A Nearly Periodic Table of Transition Elements” by James Smith and Ed Kmetko, published in the Journal of the Less-Common Metals in 1983.
Unusual properties of plutonium
- Increases in density upon melting.
- Increases in volume by 25% between two of its important phases, α and δ.
- Nonmagnetic.
- Not a good conductor of heat or electricity. Worst conductor of all metals.
- Contracts when heated in two of its phases. Becomes more compressible in delta phase.
- Very high resistivity at room temperature, increasing at lower temperatures.
- Low melting point (640°C) and an unusually high boiling point (3,230°C).
- Four oxidation states (+3 to +6) exist simultaneously in aqueous solution at an appreciable concentration.
- Unique low-symmetry crystal structures.
- Extreme sensitivity to alloying.
- Dramatic variation in mechanical properties.
- Large specific heats.
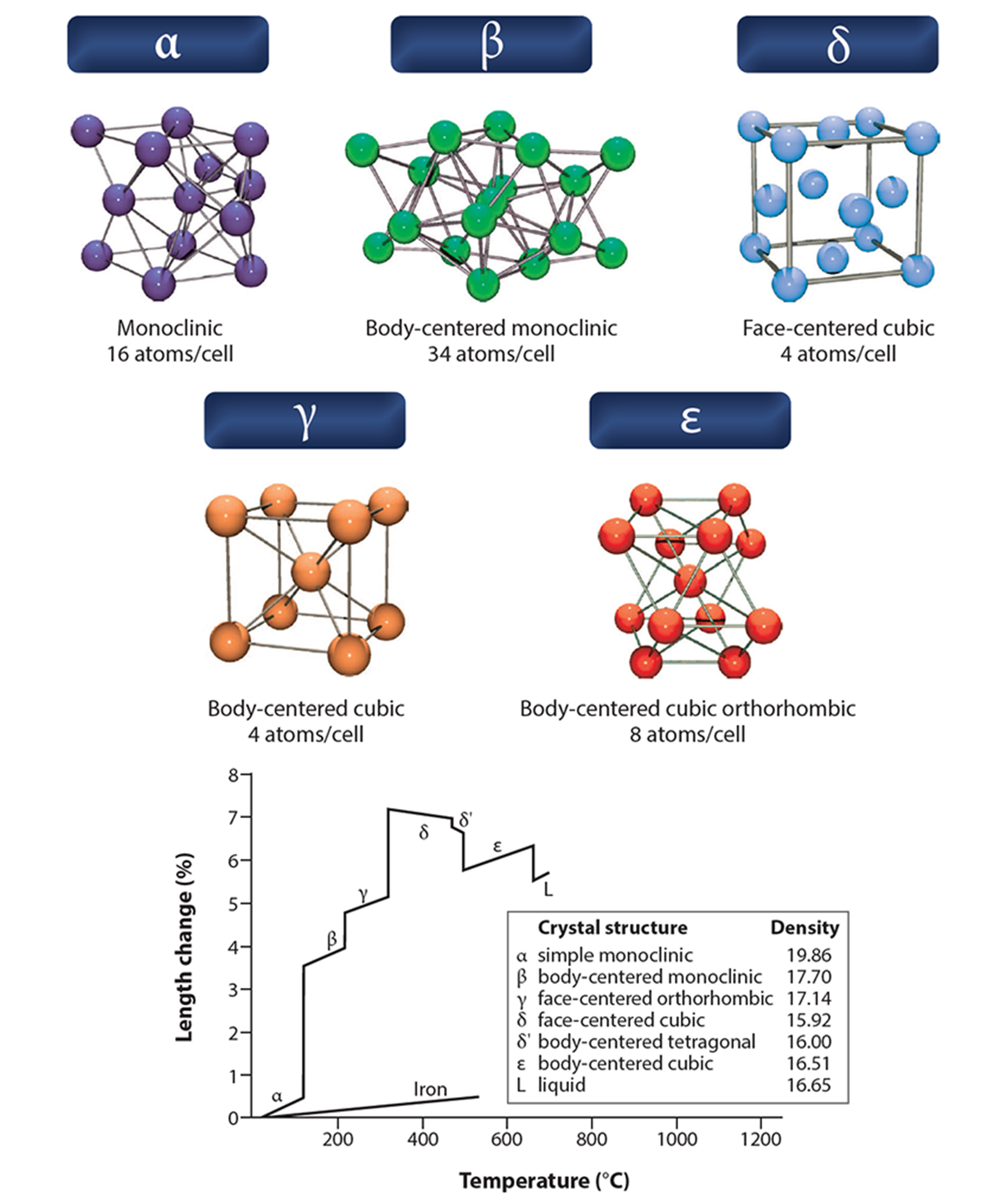
Like many oxophilic elements, plutonium metal surfaces oxidize rapidly and can burn in air, depending on conditions. This pyrophoric property, typical of actinides, resulted in two catastrophic fires in the Rocky Flats Plant in 1957 and 1969 that caused tens of millions of dollars in damage.
In 2012, the first plutonium nuclear magnetic resonance (NMR) signal was detected at LANL. Specifically, researchers observed the spin-½ nucleus plutonium-239 in PuO2 and obtained the nuclear gyromagnetic ratio. Although spin-½ nuclei are considered ideal for NMR studies (e.g., hydrogen and carbon), the plutonium signal remained elusive due to a large internal magnetic field and the large uncertainty in the nuclear gyromagnetic ratio (see H. Yasuoka, “Probing plutonium materials with magnetic resonance,” ARQ, Third Quarter 2020, p3).
Phases
Pure plutonium possesses six allotropes (α, β, γ, δ, δ', ε) between room temperature and melting at 640°C and forms a seventh (zeta, ζ) at high temperature within a limited pressure range (see figure on facing page for crystal structures). Each has significantly different properties. Note that the phases are named in order at the time of their discovery during the Manhattan Project, by crystallographers William Zachariasen and Finley Ellinger, but δ' was found around a decade later as a modified form of δ, hence its designation.
At room temperature, alpha-plutonium has an extremely high density of 19.8 g/cm3 and is as hard and brittle as cast iron (or glass). It can be alloyed with other metals (a small percentage of gallium, aluminum, or cerium) to form the roomtemperature stabilized delta form, which is soft and ductile like aluminum and can be precisely machined and welded.
Warm the unalloyed metal to 112°C, and it switches to beta phase, 10% bulkier with a density of 17.8 g/cm3. At 185°C, it changes to gamma structure, expanding another 3.5%. And at 310°C it forms the useful delta phase, expanding another 7% to become ductile (density 15.9 g/cm3, the lightest allotrope).
At 450°C, a subtle change occurs as it forms the delta prime variant, shrinking by 0.5%. A slight increase in temperature to 475°C initiates a final phase change to epsilon, with a 3% contraction.
Isotopes and uses
Plutonium has 20 identified isotopes, from mass numbers 228 to 247, inclusive. Of these, two find industrial application (238 and 239) and another six have niche uses in research. The primary decay modes of isotopes with mass numbers lower than 244 are spontaneous fission and alpha-emission, mostly forming uranium and neptunium isotopes as decay products along with a variety of daughter fission products.
Isotopes with an odd-number of nucleons are fissile with slow neutrons, notably: 239, 241, 243. In contrast, all isotopes are fissionable with fast neutrons. Mass number (A) of isotope given in blue box next to respective half-life:
236 237 half-lives = 2.8 years, 46 days
Synthesized by alpha particle irradiation of uranium-235, these isotopes have convenient half-lives to be useful for trace element studies. Plutonium radiotracers can be useful for tracking the chemical or biochemical behavior of plutonium in a complex environment, such as groundwater.
238 half-life = 87.74 years
The first isotope to be discovered, it is now used as a thermoelectric fuel source (radioisotope thermoelectric generators, RTGs) for satellites due to its high alphadecay rate. These generators are used when the probes cannot receive sufficient solar power because they have traveled too far away from the sun. Some probes that use plutonium-238 are Cassini and Galileo. It generates much more heat than plutonium-239—if it were hypothetically used in a bomb, it would create so much thermal heat that it would melt the components. It is available in isotopically pure form from neptunium-237. The use of this isotope is supported by the Accelerated Aging of Plutonium project (see Actinide Research Quarterly, Second Quarter 2002).
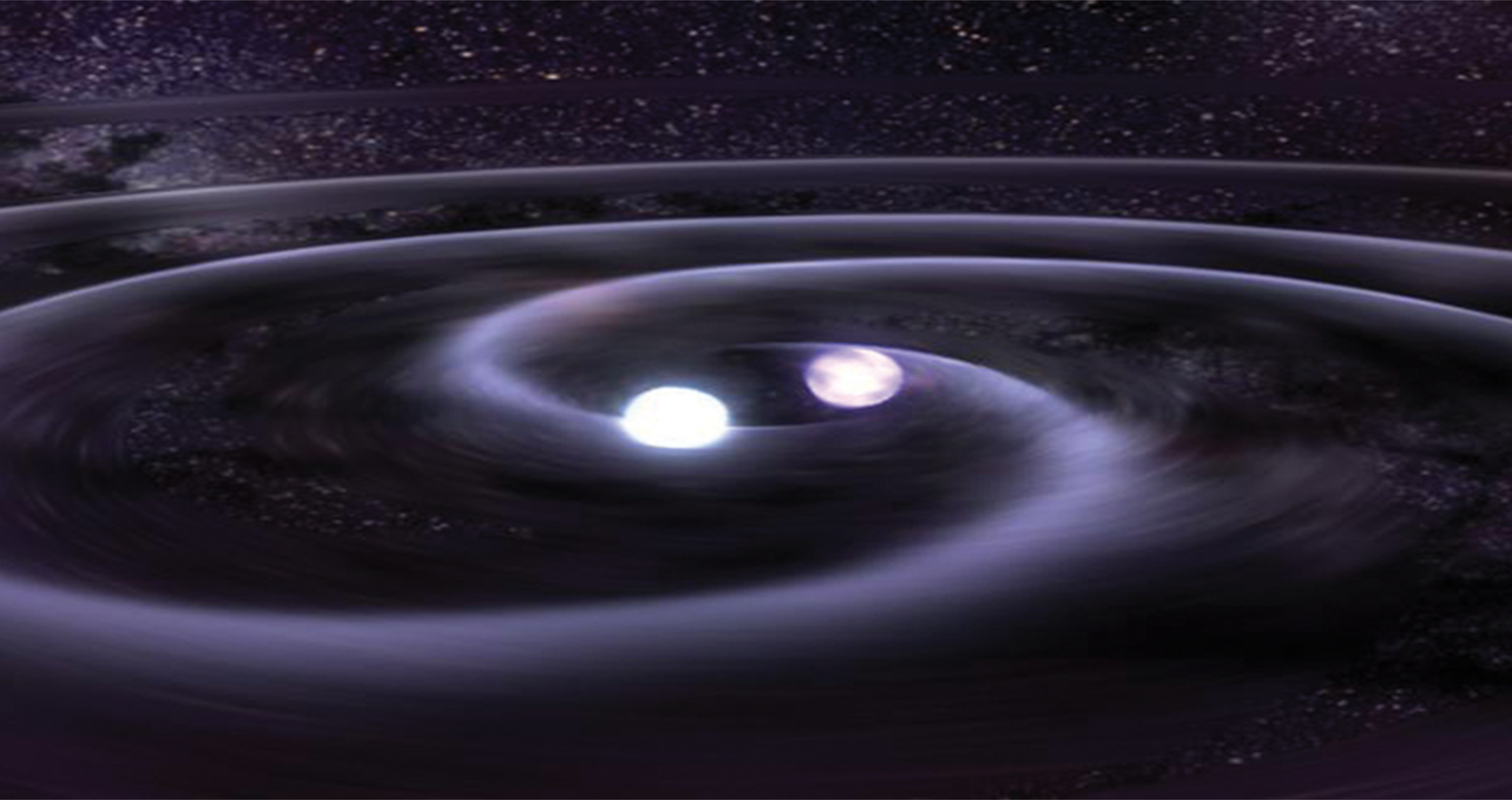
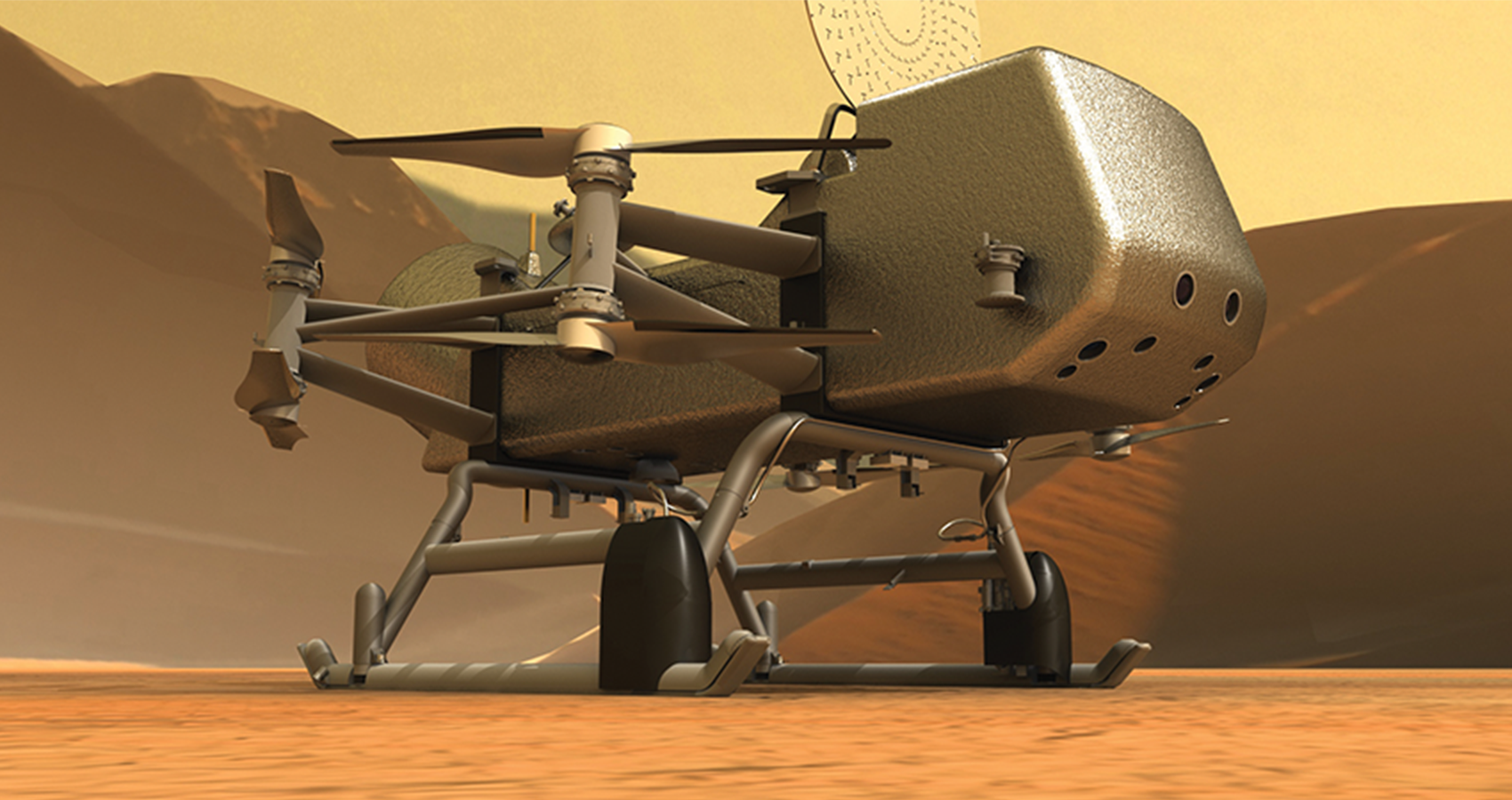
239 half-life = 24,110 years
This is the most important isotope of plutonium. The high neutron fission cross-section for both thermal and fast neutron energies enables its use as a fissile component in weapons and nuclear fuels (the other two fissile materials used for this are uranium-235 and uranium-233). It is also used to produce americium-241, commonly used in ionization-type smoke detectors. Plutonium-239 is synthesized by irradiating uranium-238 with neutrons in a nuclear reactor, then recovered via nuclear reprocessing of the fuel. Further neutron capture produces successively heavier isotopes. It has also been found to occur naturally in uranium ores, as a consequence of fission processes with uranium-235.
240 241 242 half-lives = 6,561 years, 14.3 years, 375,000 years
These isotopes are important in the nuclear fuel cycle because they are created in neutron capture reactions with lighter plutonium isotopes and have an effect on both criticality characteristics and nuclear waste properties.
Plutonium-240 is notable for its high spontaneous fission rate and is a common contaminant of samples of reactor-produced plutonium-239. Plutonium is graded based on the percentage of plutonium-240 that it contains: weapons grade (< 7%), fuel grade (7–19%), and reactor grade (> 19%). Lower grades are less suited for nuclear weapons and thermal reactors but can fuel fast reactors.
Reactor grade plutonium still poses a proliferation problem, however, as these isotopes (240 and 242) have a larger fission cross-section than uranium-235. The International Atomic Energy Agency (IAEA) conservatively classifies all isotopes of plutonium as “direct-use” material, that is, “nuclear material that can be used for the manufacture of nuclear explosives components without transmutation or further enrichment.”
244 half-life = 80,800,000 years
Along with plutonium-239, this is one of only two isotopes of plutonium that is found in nature, albeit in extraordinarily small quantities. Curiously, the official atomic weight of plutonium is defined as 244—derived from the weight of the very rare amounts of the element that occur naturally. Therefore, scientists using other plutonium isotopes have to change the atomic weight they use in calculations accordingly, a fairly unusual step! It is not significantly produced in nuclear reactors because its precursor, plutonium-243, has a short half-life and does not have the opportunity to further capture neutrons, but some is produced in nuclear explosions. It is the fourth longest-lived actinide isotope after three naturally abundant ones: uranium-235 (704 million years), uranium-238 (4.468 billion years), and thorium-232 (14.05 billion years).
It has been theorized that plutonium-244 should be present as a remnant from rapid neutron-capture process nucleosynthesis occurring at the creation of the solar system. In other words, it could be a primordial nuclide—an isotope found on Earth that has existed in its current form since before Earth was formed (there are 286 such nuclides known). Considering that all these nuclides must exist for at least 4.6×109 years, plutonium-244 (half-life 8.08×107 years) must survive 57 half-lives and therefore be reduced by a factor of 257 ≈ 1.4×1017, leaving it as the rarest of these nuclides present only in vanishingly small quantities. Geochemists have calculated that the total amount present in the Earth’s crust is just nine grams. It has been unambiguously confirmed as a galactic hitchhiker in meteorite debris and primordial plutonium remains an open research topic of continued interest.
Salvioni balance
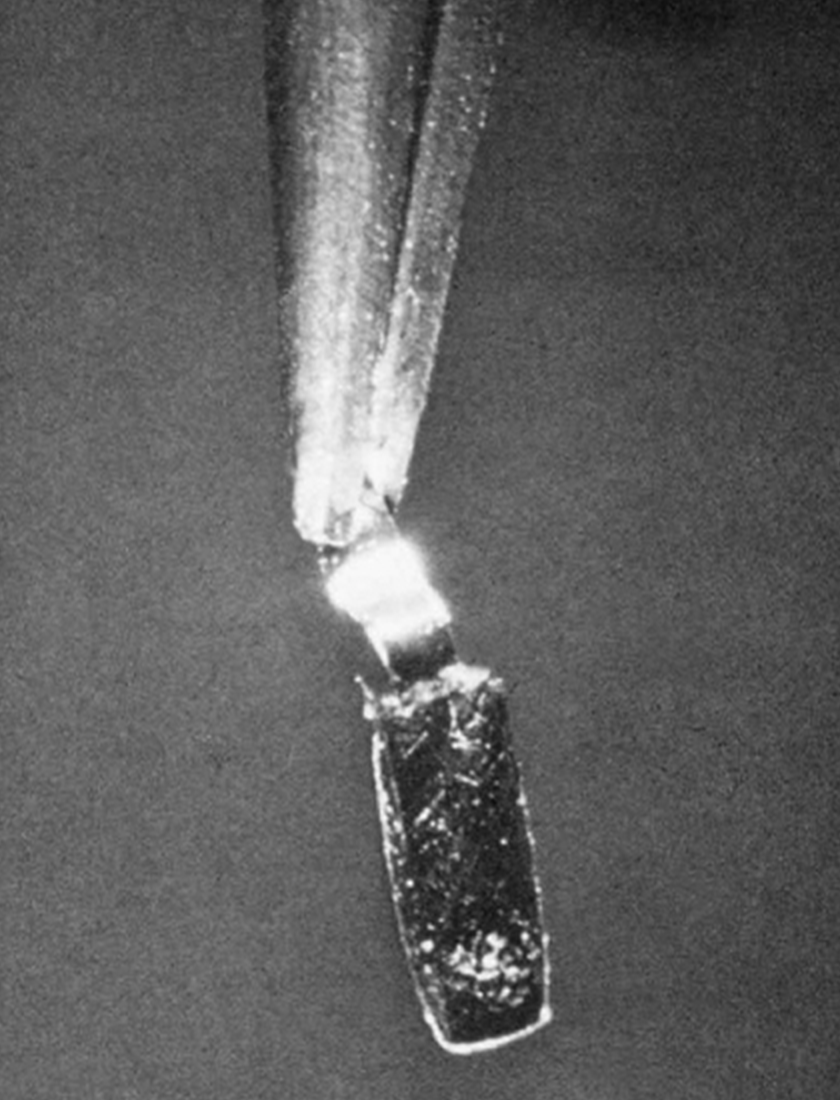
The Salvioni balance, with a sensitivity of 0.02 μg, a weighing range of 20 μg, and a capacity of 0.5 μg. It uses a quartz fiber ~0.06 mm in diameter, 12 cm in length, an adjustable stop, an aluminum cradle for the weighing pan (~200 μg) and a platinum weighing pan (~150 μg). The sample is weighed by measuring the bend in the fiber through a microscope.
See the image to the right of the first isolated sample of plutonium large enough to be weighed, September 10, 1942 (2.77 μg; 20× magnification on a platinum pan). The sample was added to the pan as a nitrate solution, which was then evaporated and heated to give the oxide, visible as a thin crust on the pan towards the bottom of the photograph.
The early studies of plutonium, when only microscopic amounts of the metal were available, required a type of chemistry that deals with quantities measured in millionths of a gram—ultramicrochemistry. Although tracer chemistry can be also used for these scales, it is limited to highly diluted substances, whereas ultramicrochemistry can be used for pure or highly concentrated solutions.
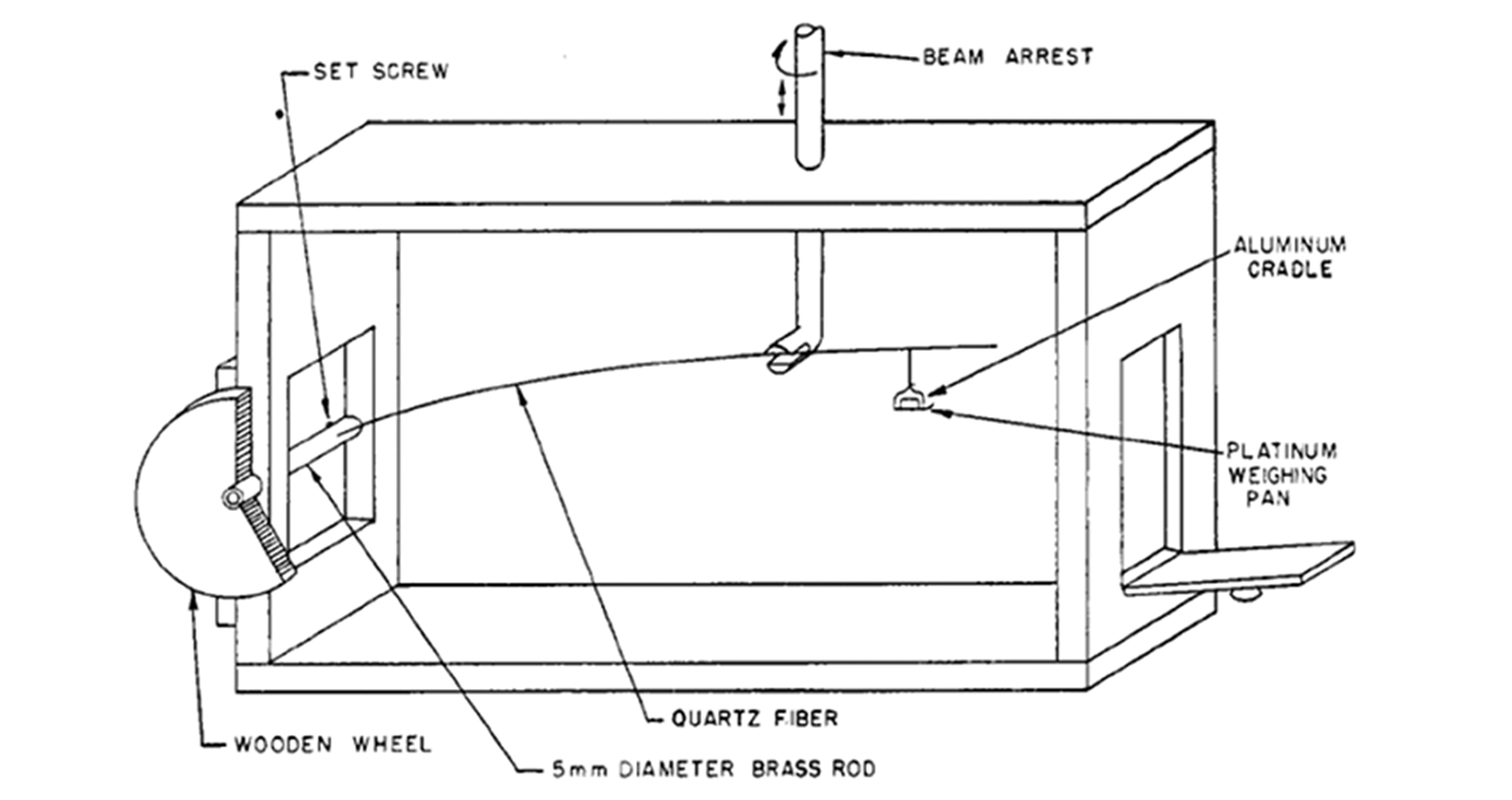
Tickling the dragon’s tail: Criticality accidents with the “Demon Core”
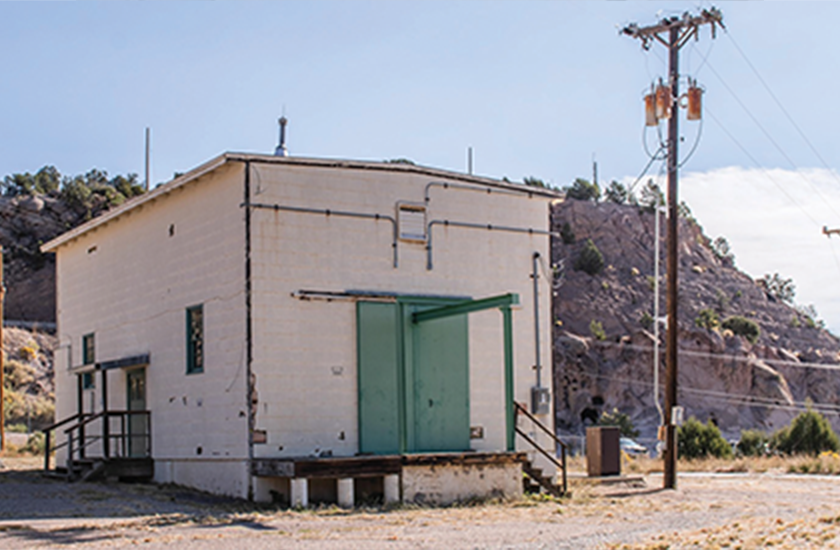
Now infamous in actinide history, two fatal criticality accidents in 1945/46 involved the same 14 lb sphere of plutonium, earning this cast the forbidding moniker “The Demon Core.” A subcritical mass, which would have been the third wartime nuclear weapon (codenamed Rufus), Manhattan Project scientists used this in experiments to measure the criticality point, i.e., the mass at which neutron flux reaches a self-sustaining level and produces fission.
It may seem one-sided to examine two high-profile American deaths compared with the tens of thousands of Japanese that were killed by the weapons. We do not ignore this fact; nonetheless, these accidents illuminate the risks that scientists freely took in the rush to produce the bomb, and offer visceral insights into the challenges of studying the nuclear properties of plutonium.
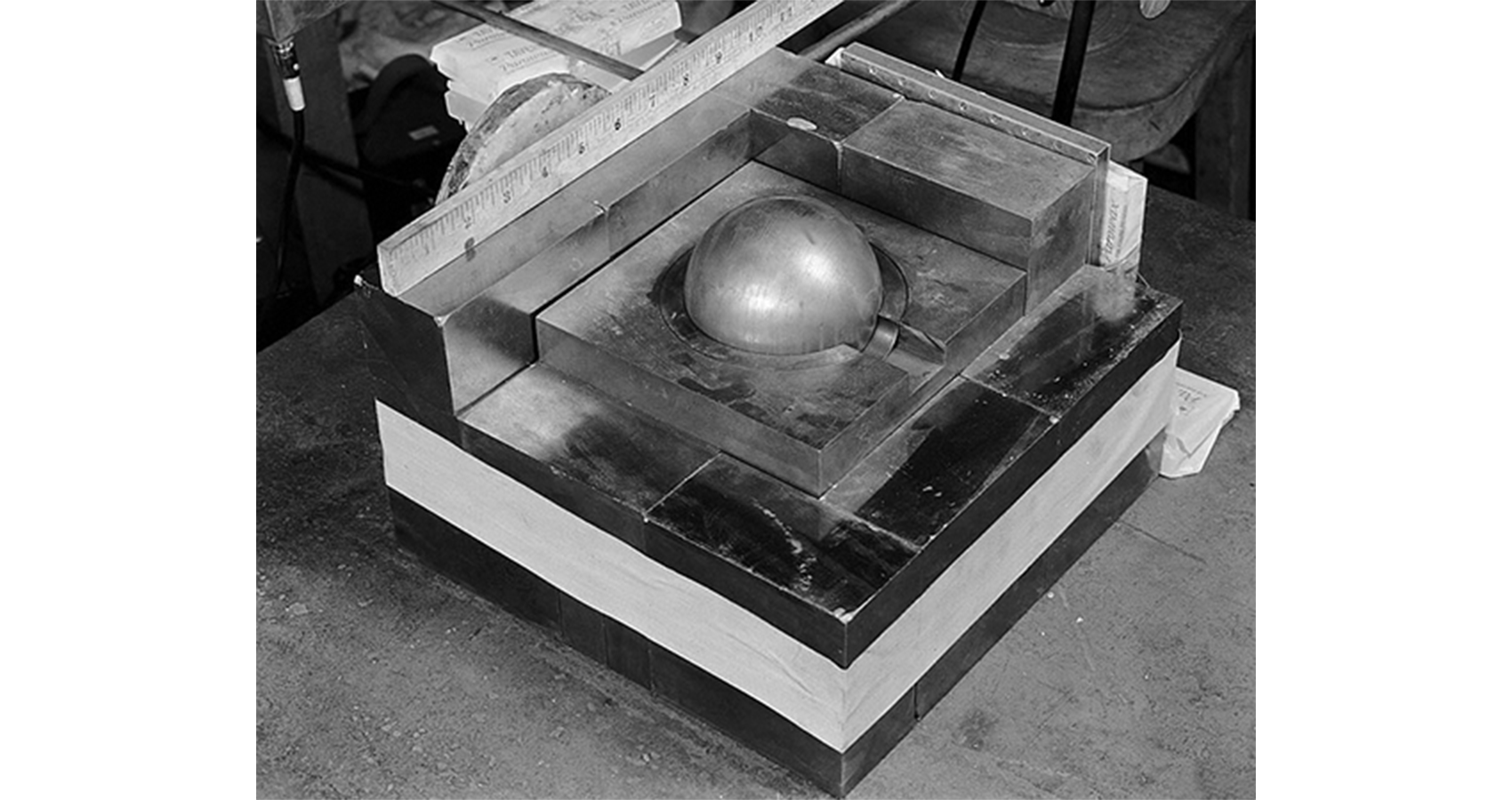
Harry Daghlia
The first accident occurred on August 21, 1945, just two days after the cancellation of Demon Core’s bombing run and twelve days after the bombing of Nagasaki. Harry Daghlian was using tungsten carbide bricks to intensify neutron radiation in the core. By using them as mirrors to bounce the neutrons back into the mass, Daghlian was trying to incrementally push the mass closer to criticality. The idea was to approach this point but not step over the limit. Flirting with the chance of a nuclear chain reaction, these type of criticality experiments became known as “tickling the dragon's tail,” based on a remark by Richard Feynman.
Working alone at night, Daghlian had returned after he had nearly obtained his measurement during the day. The core approached supercriticality, so he picked up a brick to end the experiment but unfortunately for him he had slippery fingers. As he was taking the brick away he accidentally dropped it. It landed on top of the plutonium sphere—a flash of blue light (Cherenkov radiation) and wave of heat told him that the core had gone critical. He reached in to snatch the brick away and prevent damage to the valuable core, but it was too late and he received a lethal dose, later estimated at 5,100 mSv. After 25 days in hospital he was dead, aged 24, from anemia and organ failure.
Louis Slotin
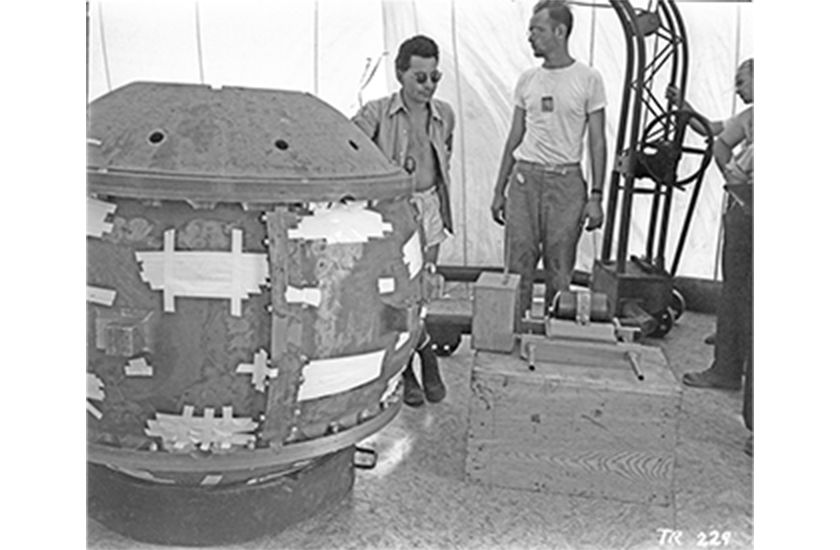
Slotin, a friend of Daghlian’s and his team supervisor who had tended to him every day in hospital, continued his work on the core during the following year, using a different method with a pair of nine-inch beryllium hemispheres as reflective material (named “tampers”) instead of tungsten carbide. Lowering the upper hemisphere manually, a small gap was kept to prevent it going critical. Instead of using spacers as fail-safes, however, he held a screwdriver blade to prop up the dome and prevent it closing. Fermi reportedly told Slotin and others they would be “dead within a year” if they continued performing the test in that manner.
On May 21, 1946, Slotin was demonstrating the technique to a group at Omega Site ahead of its use in the Operation Crossroads Pacific nuclear tests. The accident happened when the screwdriver Slotin was holding slipped a fraction of an inch, allowing the two hemispheres to close, exposing the scientists to approximately a half second of intense radiation.
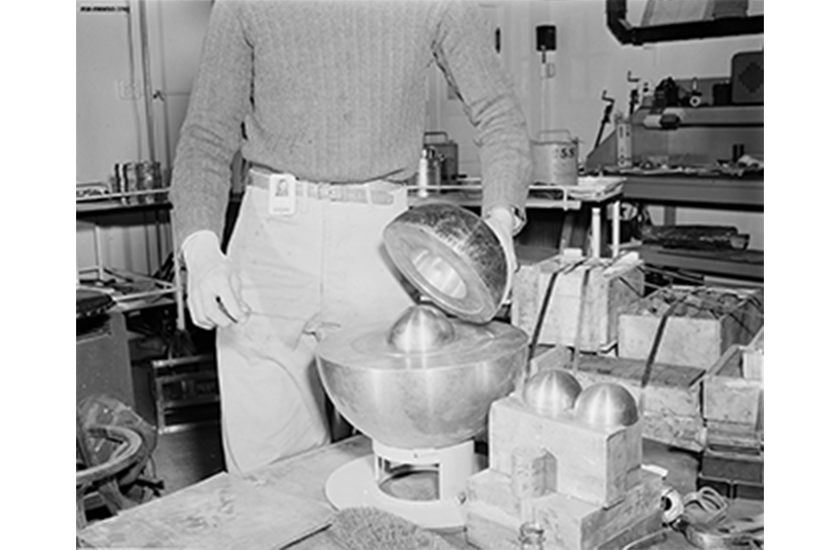
Everyone in the room received a massive dose of radiation, however Slotin was the only one to have a fatal dose. Nevertheless, fully aware of this likelihood, he still kept his wits and quickly disassembled the device, thereby likely saving the lives of seven others nearby. He also recorded exactly where everyone had been standing using chalk and thus calculated their exact radiation dose. He died within nine days— doctors described his internal injuries as “three dimensional sunburn”—in the same hospital as Daghlian. He was 35.
These accidents led to a safety overhaul—after Slotin’s death, all hands-on criticality experiments ended at Los Alamos and were replaced by the Los Alamos Critical Experiments Facility. A memo was written shortly after the accident that recommended that future experiments should use remote controls and make “more liberal use of the inverse-square law.” Instead of being used in the Pacific tests, the Demon Core was instead melted down and re-integrated into the US nuclear stockpile.
LANL has preserved the location where this accident happened, now known as the Slotin Building . It is on LANL property, but part of the Manhattan Project National Historical Park and visitors can access it via guided tours on specific dates.
Plutonium, memorialized
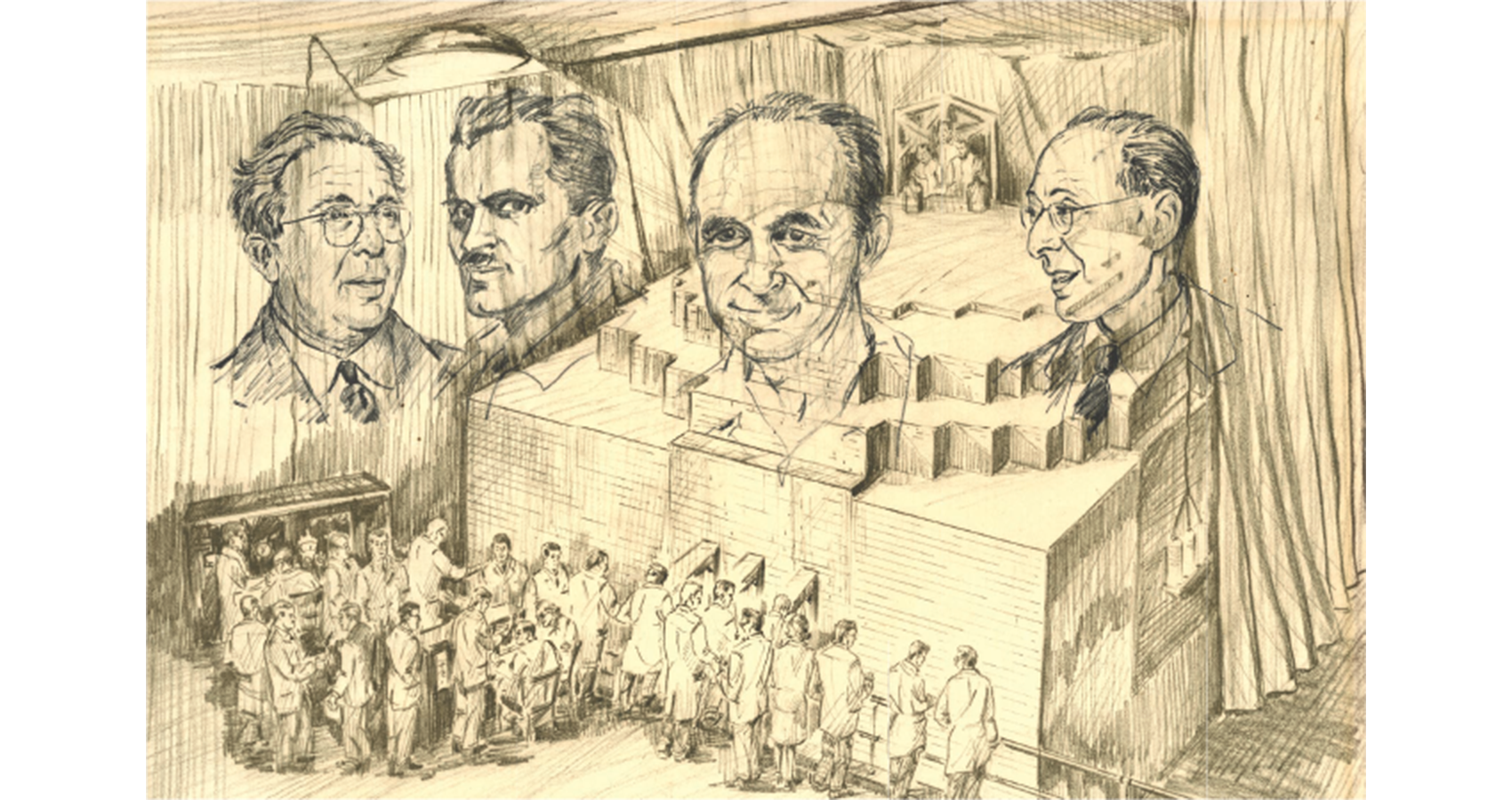
Artist Leo der Vartanian depicted Chicago Pile-1 in this undated lithograph. Remarkably, he used ink containing a portion of the actual neutron moderator graphite from the piles—it is thought that this special ink was reserved for the enlarged faces. These four prominently featured scientists are, from left: Leo Szilard (conceived the nuclear chain reaction idea in 1933), Arthur Compton (head of the Chicago Metallurgical Laboratory and overseer of the CP-1 project), Enrico Fermi (leader of the team that designed and built CP-1), and Eugene Wigner (leader of the team that designed production nuclear reactors to convert uranium into weapons grade plutonium).
The framed lithographs were awarded by Argonne National Laboratory to distinguished employees. An unknown donor gave one of these copies to the National Security Research Center, which is the classified library at Los Alamos National Laboratory, where it is displayed among other legacy items from our Lab’s fascinating history.
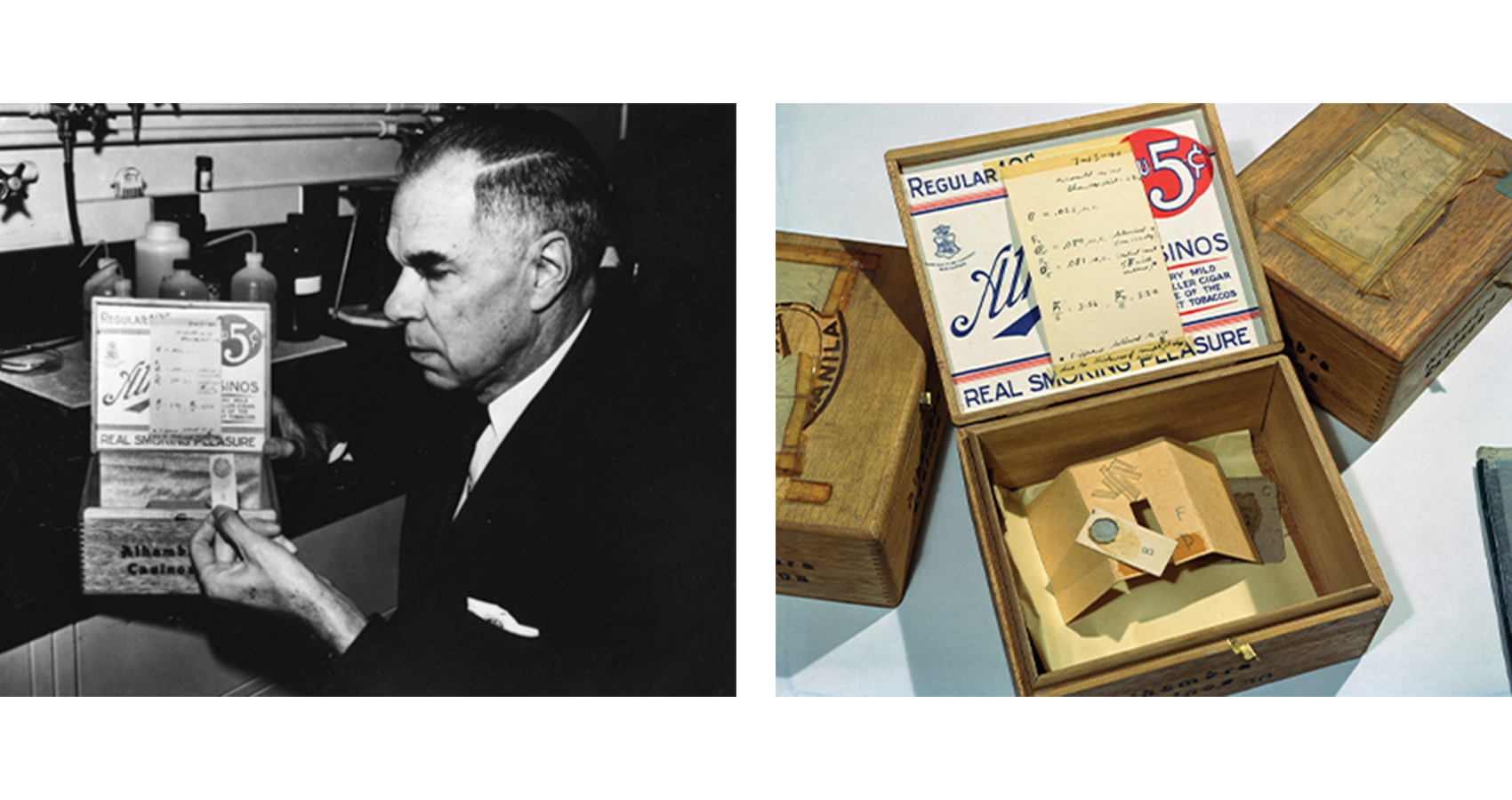
On the 25th anniversary of the discovery of nuclear fission of plutonium-239 (March 28, 1966), Glenn Seaborg and Emilio Segrè presented the original plutonium sample and its cigar box container to the Smithsonian Institution. The note on the front of the box reads: “Very valuable sample. Do not disturb! J.W. Kennedy.”
The sample comprises an original platinum dish (measured two-thirds of an inch across and half an inch in depth) containing 0.25 μg of plutonium-239, preserved with a protective layer of Duco Cement glued to a piece of cardboard. The cigar box belonged to the legendary Berkeley chemist G.N. Lewis, who was Seaborg’s supervisor from 1937–39.
Nobel Prizes
No Nobel prizes were given directly for the discovery of plutonium, for the reason that one of its main uses was in warfare. Nevertheless, many of the essential developments which directly led to it were recognized:
- Irène and Frédéric Joliot-Curie, Chemistry, 1935 “in recognition of their synthesis of new radioactive elements.”
- James Chadwick, Physics, 1935 “for the discovery of the neutron.”
- Enrico Fermi, Physics, 1938 “for his demonstrations of the existence of new radioactive elements produced by neutron irradiation, and for his related discovery of nuclear reactions brought about by slow neutrons.”
- Ernest O. Lawrence, Physics, 1939 “for the invention and development of the cyclotron and for results obtained with it, especially with regard to artificial radioactive elements.”
- Otto Hahn, Chemistry, 1944: “for his discovery of the fission of heavy nuclei.”
- Glenn T. Seaborg, Edwin McMillan, Chemistry, 1951 “for their discoveries in the chemistry of the transuranium elements.”
The Manhattan Project (MP) recruited several of the finest scientists in the world. Many of the most prominent figures were either already Nobel laureates or would go on to earn the award for work outside of plutonium (MP scientists in bold):
- Niels Bohr, Physics, 1922 “for his services in the investigation of the structure of atoms and of the radiation emanating from them.”
- James Franck, Gustav Hertz, Physics, 1925 “for their discovery of the laws governing the impact of an electron upon an atom.”
- Arthur H. Compton, Physics, 1927 “for his discovery of the effect named after him.”
- Harold Urey, Chemistry, 1934 “for his discovery of heavy hydrogen.”
- Percy Bridgman, Physics, 1946 “for the invention of an apparatus to produce extremely high pressures, and for the discoveries he made therewith in the field of high pressure physics.”
- Felix Bloch, Edward Purcell, Physics, 1952 “for their development of new methods for nuclear magnetic precision measurements and discoveries in connection therewith.”
- Emilio Segrè, Owen Chamberlain, Physics, 1959 “for their discovery of the antiproton.”
- Eugene Wigner, Physics, 1963 “for his contributions to the theory of the atomic nucleus and the elementary particles, particularly through the discovery and application of fundamental symmetry principles.”
- Maria Goeppert Mayer, J. Hans D. Jensen, Physics, 1963 “for their discoveries concerning nuclear shell structure.”
- Richard Feynman, Sin-Itiro Tomonaga, Julian Schwinger, Physics, 1965 “for their fundamental work in quantum electrodynamics, with deep-ploughing consequences for the physics of elementary particles.”
- Hans Bethe, Physics, 1967 “for his contributions to the theory of nuclear reactions, especially to his discoveries concerning the energy production in stars.”
- Luis Alvarez, Physics, 1968 “for his decisive contributions to elementary particle physics, in particular the discovery of a large number of resonance states, made possible through his development of the technique of using hydrogen bubble chamber and data analysis.”
- Aage Bohr, Ben Mottelson, Leo James Rainwater, Physics, 1975 “for the discovery of the connection between collective motion and particle motion in atomic nuclei and the development of the theory of the structure of the atomic nucleus based on this connection.”
- Val Fitch, James Cronin, Physics, 1980 “for the discovery of violations of fundamental symmetry principles in the decay of neutral K-mesons.”
- Norman Ramsey, Physics, 1989 “for the invention of the separated oscillatory fields method and its use in the hydrogen maser and other atomic clocks.”
- Frederick Reines, Clyde Cowan, Physics, 1995 “for pioneering experimental contributions to lepton physics.”
- Roy Glauber, Physics, 2005 “for his contribution to the quantum theory of optical coherence.”
Nobel Peace Prizes for disarmament and non-proliferation:
- Philip Noel-Baker, 1959 “for his longstanding contribution to the cause of the disarmament and peace.”
- Linus Pauling, 1962 “for his fight against the nuclear arms race between East and West.”
- Eisaku Sato, 1974 “for his contributions to stabilize conditions in the Pacific Rim area and for signing the Nuclear Non-Proliferation Treaty.”
- Alva Myrdal, Alfonso Garcia Robles, 1982 “for their work for disarmament and nuclear and weapons-free zones.”
- International Physicians for the Prevention of Nuclear War, 1985 “for spreading authoritative information and by creating awareness of the catastrophic consequences of nuclear war.”
- Joseph Rotblat and Pugwash Conferences on Science and World Affairs, 1995 “for their efforts to diminish the part played by nuclear arms in international politics and, in the longer run, to eliminate such arms.”
- International Atomic Energy Agency (IAEA) and Mohamed ElBaradei, 2005 “for their efforts to prevent nuclear energy from being used for military purposes and to ensure that nuclear energy for peaceful purposes is used in the safest possible way.”
- International Campaign to Abolish Nuclear Weapons (ICAN), 2017 “for its work to draw attention to the catastrophic humanitarian consequences of any use of nuclear weapons and for its ground-breaking efforts to achieve a treaty-based prohibition of such weapons.”
Acknowledgments
I would like to thank Franz Freibert and David Clark for useful discussions relating to plutonium, and Alan Carr and John Moore for supplying historical photos from the archives here at LANL. I am also grateful to the Emilio Segrè Visual Archives for additional images.
Further reading:
- J. Bernstein, “Plutonium: A History of the World’s Most Dangerous Element,” The Joseph Henry Press, Washington, DC, 2007.
- J. Bernstein, “Hitler’s Uranium Club,” Copernicus, New York, 2001.
- G.T. Seaborg, E. Seaborg, “Adventures in the Atomic Age,” Farrar, Straus and Giroux, New York, 2001.
- D.C. Hoffman, A. Ghiorso, G.T. Seaborg, “The Transuranium People: The Inside Story,” Imperial College Press, London, 2000.
- “Plutonium Handbook,” Second Edition, Eds. D.L. Clark, D.A. Geeson, R.J. Hanrahan, Jr., American Nuclear Society, La Grange Park, Illinois, 2019.
- A. Michaudon, “From alchemy to atoms: The making of plutonium,” Los Alamos Science, 2000, 26, 62.
- S.S. Hecker, “Plutonium—An element at odds with itself,” Los Alamos Science, 2000, 26, 16.
- S.S. Hecker, “Plutonium: Manhattan Project to today,” ARQ, 2019, Fourth Quarter, 14.
- G.T. Seaborg “Source of the actinide concept,” ARQ, 1997, Second Quarter, 1, reprinted in 2020, First Quarter, 4.
- J.C. Martz, F.J. Freibert, D.L. Clark, “The taming of plutonium: Plutonium metallurgy and the Manhattan Project,” Nucl. Technol., 2021, 207, S266.
Manhattan Project long reads, as recommended by Lab historian Alan Carr:
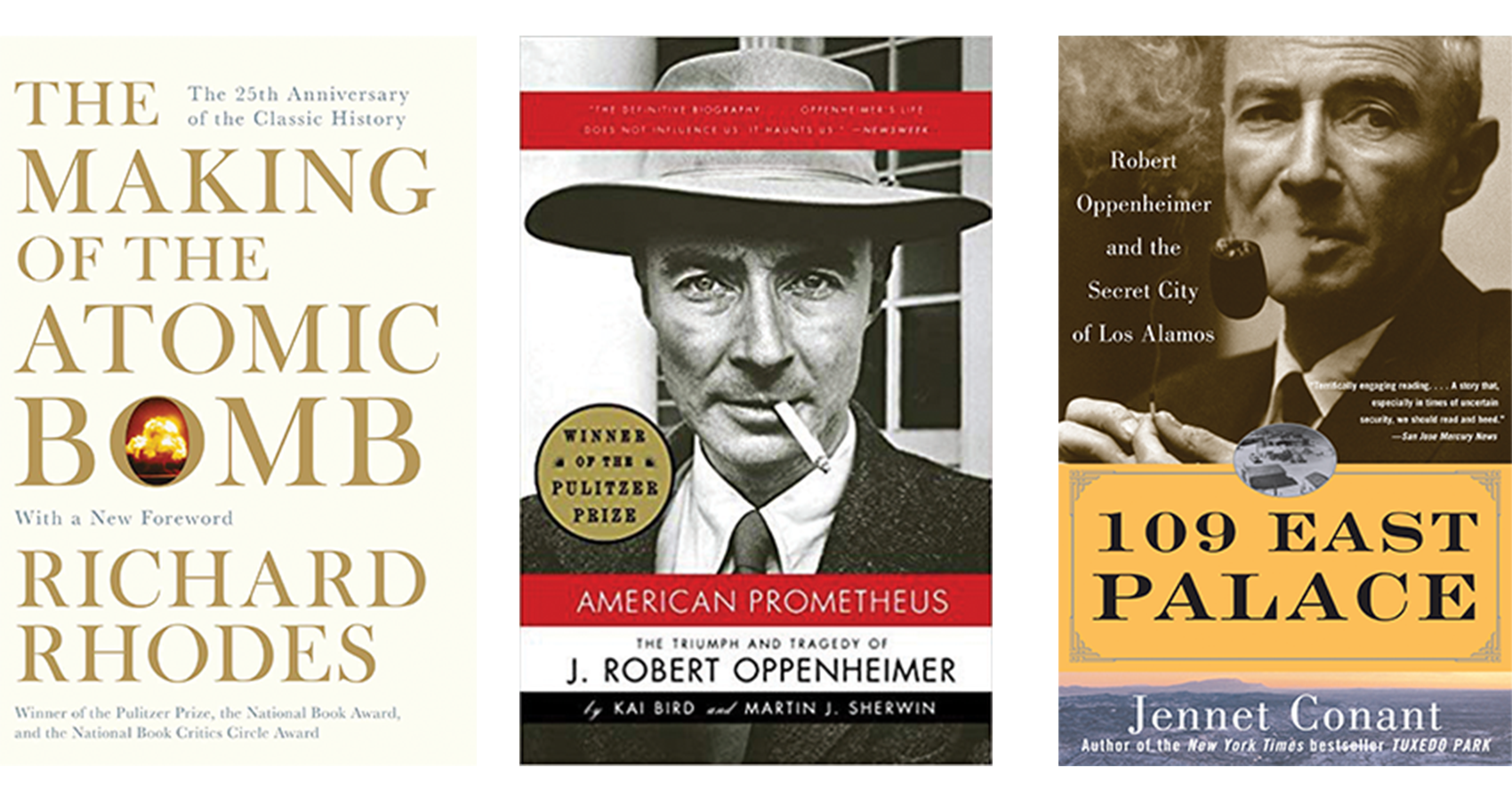
Middle: The Pulitzer Prize-winning definitive biography of Oppenheimer. Currently being adapted into a Hollywood movie by Christopher Nolan: “Oppenheimer” to be released in July 2023.
Right: A popular and readable account of Project Y that focuses more on the social aspects of Los Alamos largely from the perspective of Dorothy McKibbin, the secretary who ran the Lab’s Santa Fe office.
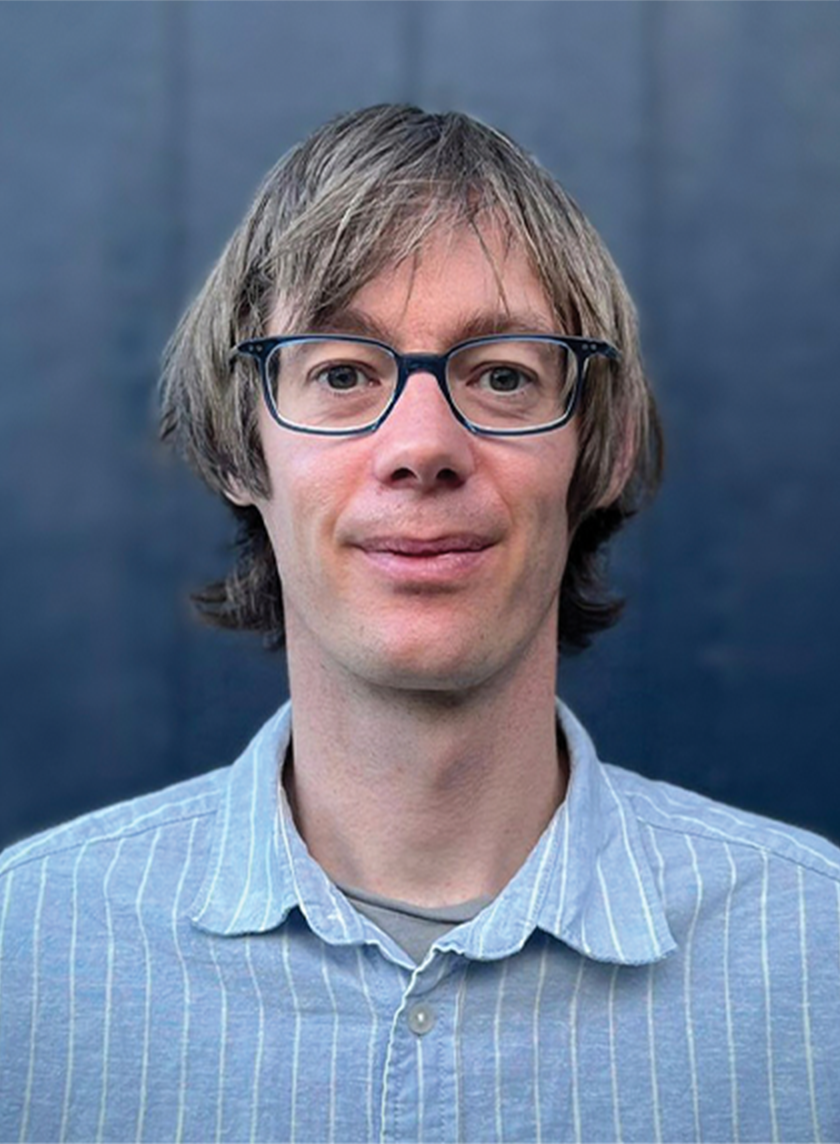
Owen Summerscales
Owen Summerscales is the current editor of Actinide Research Quarterly. He received his MChem in chemistry from Oxford University in 2003 and a DPhil in inorganic uranium chemistry from the University of Sussex in 2007. He has worked as a scientific editor since 2016.