I do my best work when I maintain an uncomfortable balance between two things: studying fundamental nuclear astrophysics—what happens in stars—and applying nuclear physics to answer mission-relevant questions—understanding what happens during nuclear explosions and within nuclear reactors. I say “uncomfortable” because either of these things could keep me busy night and day. I say “best” because each provides insight and tools to help the other advance—this combination is what makes science at Los Alamos unique and positions the Lab to do work that can be accomplished nowhere else.
Sixteen years ago, I came to Los Alamos to work at the Los Alamos Neutron Science Center, or LANSCE, because I was attracted to the idea of using neutrons to study nuclear physics, and LANSCE has neutrons, lots of neutrons. Not many other places do. So, when an opportunity came to me, via my mentor and colleague René Reifarth, to join the Lab as a post doc, it was an easy decision to make.
I wanted to study nuclear physics using neutrons and Los Alamos has neutrons. Not many places do.
Pretty much all the elements, once you get past helium, come from stars. Lighter elements are formed in stars by nuclear fusion, whereby two nuclei fuse into one larger nucleus. Heavier elements are formed in stars by neutron capture, whereby a nucleus absorbs neutron after neutron until, by way of a process called beta decay, the nucleus gains a proton and turns into the next element on the periodic table. But how… exactly? My career so far has been spent working to understand how different combinations of elements and particles react when they are put together inside nuclear weapons, inside nuclear reactors, or inside stars—we, as scientists, need to understand the reactions that are happening.
A free neutron is a pesky, ill-behaved particle. Unlike neutrons that are bound in an atomic nucleus, a free neutron only exists for about 15 minutes before beta decaying into a proton, an electron, and an antineutrino. During those 15 minutes it can cause all kinds of trouble, ambling along and interacting at a nuclear level with almost whatever it finds. Because the neutron is electrically neutral, there is no electric field to push it away from an atom. These encounters with atomic nuclei can have a variety of consequences. If the neutron has a lot of energy, it can knock another neutron (or several) out of the nucleus, changing the isotope of the nucleus. Or, the neutron can just bounce off the nucleus, carrying on in another direction much like a ball on a billiard table. Finally, sometimes a neutron is captured by the nucleus, making the nucleus into a new isotope of the same element, just a bit heavier than before. For example, an iron-58 atom may capture a neutron to become iron-59.
A free neutron is a pesky, ill-behaved particle.
Neutron capture, with all its complexities, is how heavy elements are synthesized within stars. But neutrons are also a powerful tool for probing and understanding physical systems here on Earth. For example, through neutron diffraction—or the scattering of neutrons off a sample of material—the atomic or magnetic structure of the material can be inferred. In addition, because they can turn stable materials into radioactive ones, neutrons can be used to create certain important radionuclides such as molybdenum-99, used in medical imaging, or cobalt-60, used in cancer treatment. Neutron radiography is another important application, where neutrons can be used to penetrate a container allowing scientists to peer inside, much like x-rays, except neutrons can easily get through materials that x-rays can’t. It’s not good for looking inside people, but very good if you want to know what is inside a steel cask.
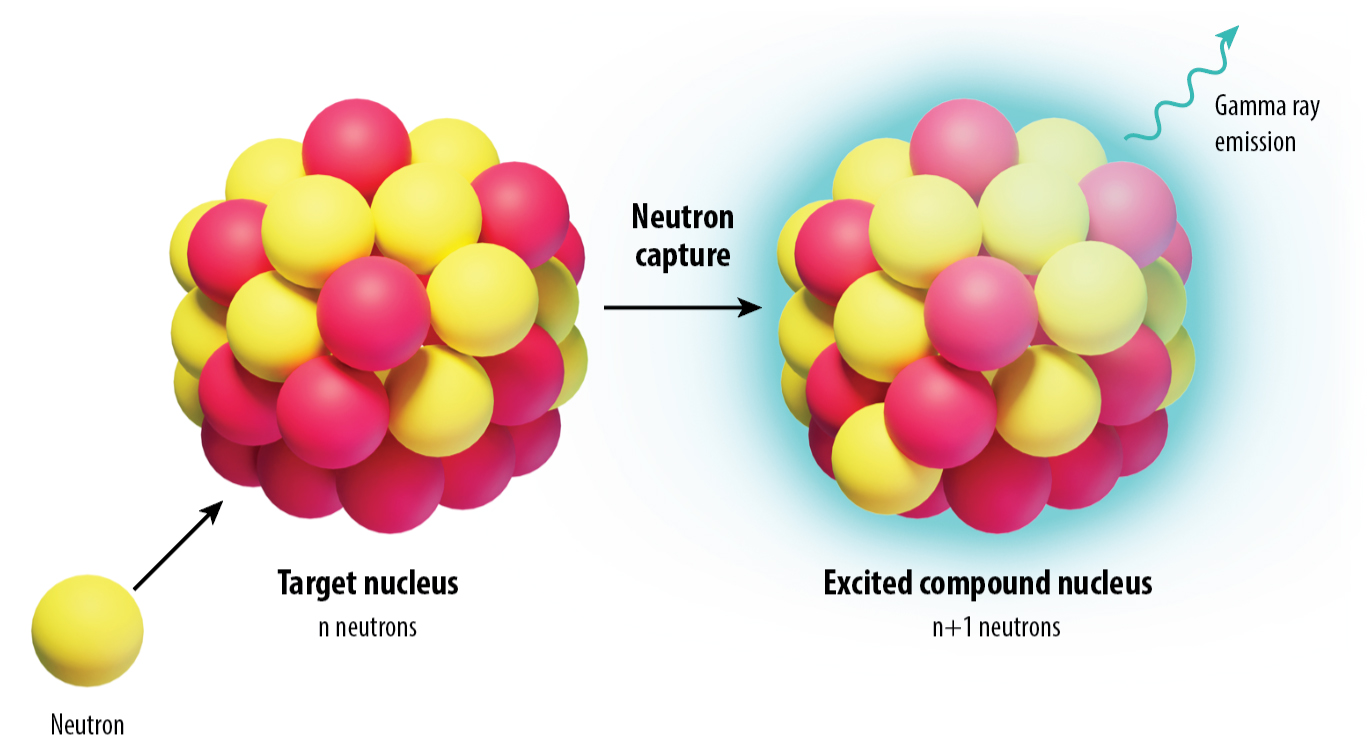
Learning to DANCE
The first experiment I worked on at LANSCE, a project I still work on, is the Detector for Advanced Neutron Capture Experiments, or DANCE. On the outside, the apparatus is a hollow aluminum sphere with a lot of wires coming out. On the inside is an array of 160 specially designed detectors, each consisting of a single crystal of barium fluoride. The crystals kind of look like 8-pound diamonds, if your gem master got lazy and only cut a few facets into each one. There is a hole through the detector array where neutrons can enter the sphere and interact with a sample—just a few milligrams placed at the center of the sphere—of whichever isotope is being studied. The only thing that varies when I am studying what happens inside stars, inside nuclear weapons, or inside nuclear reactors is which isotope makes up the sample in the middle.
Some neutron-capture products are stable while others are short-lived, but in either case the reaction makes the atom momentarily too heavy. It’s only off by about 0.001 percent, and that little bit gets emitted as electromagnetic energy. We measure the emitted energy—that’s what DANCE is uniquely suited to do. When the material under study captures a neutron within the DANCE sphere, the nucleus is excited and will then de-excite by emitting gamma rays, which get detected by the barium fluoride crystals.
The size of the signal from each detector indicates the energy of the gamma ray detected by that crystal. That energy tells us about the neutron-capture reaction that took place; that is the real nuclear physics underlying these studies. The emitted energy is unique for every possible reaction—nuclei throw off different-sized chunks of energy depending on the reaction, and that’s how we determine which reaction took place.
The likelihood of a given isotope capturing a neutron is referred to as the reaction rate for that isotope. The reaction rate allows us to calculate the rate at which neutrons are lost to the reaction and the corresponding rate at which the resulting neutron-enriched nuclei are created and do whatever they do next—whether that be nuclear fission, radioactive decay, or another neutron capture. Where things get tricky, and where DANCE is useful, is when the nucleus is a short-lived species.
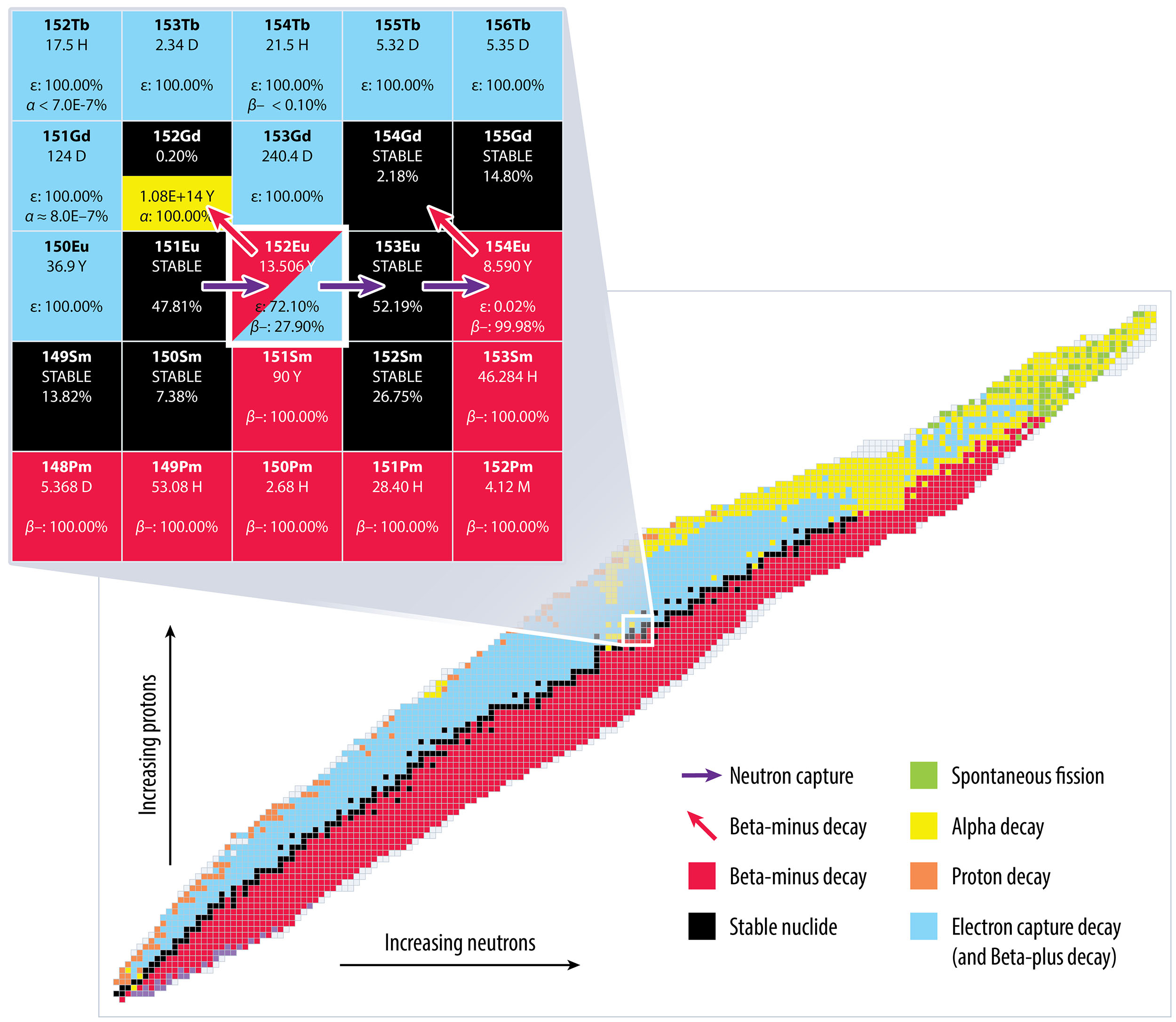
This section of the Table of Nuclides (callout) shows how the processes of gaining a neutron via neutron capture (purple arrows), and gaining a proton via beta decay (red arrows), drive the evolution of elements. In this example, gadolinium (Gd)-152 and Gd-154, both stable isotopes found in meteorites, can be traced back to europium (Eu)-152. By quantifying the ratio of Gd-152 to -154 in meteorites on Earth, and combining that information with experimental Eu reaction rate data, scientists can learn about the physical conditions within the stars where the elements were formed.
Our experimental knowledge has typically been limited to stable isotopes, but many of the most interesting (and revealing) reaction rates are on unstable isotopes. Because of the efficiency of DANCE and the intensity of the LANSCE neutron beams, we can push measurements to isotopes with half-lives as short as a couple months. DANCE measures the characteristic energy released in the reaction, so it can differentiate between the abundant gamma rays emitted by decay and those emitted in response to neutron capture. Past efforts were either limited by low-intensity neutron sources or detector arrays without DANCE’s efficiency or discriminating power.
Experiments with instruments like DANCE are only part of the neutron-capture story. We work very closely with theoreticians who model the reactions that we study, and with astrophysical modelers who use our data in stellar- and explosive-burning models to predict the abundances of isotopes in the cosmos. Our measurements help the theorists refine their models, and their model outputs help determine the need for future measurements. Through collaborative iteration we are learning the physics of both the nuclei and their environments; this is how we build our understanding of nuclear reactions, whether they are happening inside a star or somewhere here on Earth.
I found my "uncomfortable balance" early on, and when I first got here, my DANCE work was split between two different projects. One involved neutron-capture studies on plutonium-240 and -242 for the Department of Energy’s Office of Nuclear Energy’s Advanced Fuel Cycle Initiative. Essentially, I was helping design next-generation “fast” nuclear reactors, which run with a higher-energy neutron spectrum than older thermal-water reactors. The other project was to measure a key reaction on europium-152 (Eu-152), a relatively short-lived isotope with a half-life of 13 years. Neutron capture by Eu-152 affects gadolinium-152 and -154 abundances, which can be measured in meteorites—bits of stardust fallen to Earth—revealing clues about astrophysical nucleosynthesis processes. So, a solid understanding of the reaction rates for neutron capture on Eu-152 informs our understanding of the late middle-ages of stars a bit larger than our sun.
Several other recent or ongoing projects help illustrate the breadth of applications and techniques that can be done with DANCE. The first is a study of neutron capture on uranium-233, which is important for the national Nuclear Criticality Safety Program. This is a challenging isotope, not only because it is radioactive, but because neutrons can cause it to undergo fission—the principle underpinning nuclear weapons and nuclear reactors—which can obscure the capture signal because fission also creates a spectrum of gamma rays. Esther Leal Cidoncha, a postdoc working with me who leads this project, learned that by using DANCE together with a neutron detector array to identify fission events that took place during the measurement, those fission events can be isolated and removed from the signal in analysis. This only works with an instrument like DANCE because it measures both the number of the gamma rays emitted as well as their energy spectrum.
The second project was a study of thulium-171, an isotope with about a two-year half-life. The sample used in this study was produced by collaborators in Europe—often producing a physical sample of a radioactive material is the difficult part—and shipped to us at LANSCE for the measurement. Thulium is of interest because it was used as a radiochemical detector during the days of the nuclear testing program and quantifying its various reaction rates is important for our continued mission for the National Nuclear Security Administration’s Defense Programs.
Finally, we recently completed a neutron-capture study on germanium isotopes, an effort that was led by collaborators at Louisiana State University. The production of elements from nickel through zirconium within stars proceeds mainly via neutron capture. Our work pinned down the reaction rates for these elements in stars roughly ten times larger than the sun, which have been suggested as the main sources of these elements throughout the universe. These direct measurements on “easy” isotopes are often some of the only windows we have into the astrophysical processes that make all the heavy elements—they are an island of confidence in a sea of uncertainty.
By hook or by crook
I often joke that I determine neutron-capture reaction rates “by hook or by crook”—meaning if I can’t do it with one method then I’ll find another one. DANCE is a great way to measure reaction rates, but for some radioactive isotopes, it’s simply not possible. Either the decay radiation overwhelms the instrument, or the isotope of interest, which could have a half-life of as little as seconds, decays away before we can complete the measurement.
Based on some of the physics we learned from DANCE measurements on uranium-238, I had the idea to use a transfer reaction—where the neutron is not free but moves from one bound nucleus to another—to study how an excited nucleus gives off energy and to inform neutron capture. Certain stable, light isotopes, like deuterium or lithium-7 make good targets while a beam of heavy, short-lived isotopes can be used to study other aspects of the same physics. To pursue this transfer-reaction idea, my team and I designed and built a scintillator array. We named the array Apollo and took it to the ATLAS linear accelerator at Argonne National Lab, near Chicago.
ATLAS has a heavy ion accelerator, meaning that unlike LANSCE, which makes beams of protons and neutrons, ATLAS makes beams of entire elements like iron, zirconium, and xenon. We used these to measure the gamma rays emitted when charged-particle reactions donate a neutron to the final nucleus. This did, and continues to, reveal important information about how a nucleus emits energy. But to connect the Apollo data back to neutron capture requires a lot of theory—to model the charged particle reaction, and also to transform the data into useful information about neutron-induced reactions, which can be a limitation.
Where both DANCE and DICER have measured the same sample, we have exquisitely detailed information.
Another idea came from my colleague Paul Koehler, who suggested neutron transmission might be used to measure some of the same reaction probabilities as neutron capture can, but without a key constraint of DANCE. For DANCE, the sample sits inside the detector array, so if it makes a lot of gamma rays, DANCE will see them all. At some point, even with as good as DANCE is, there is simply too much decay to still see a signal. Paul’s idea was to do a measurement where the sample and the detector are separated by 10 meters or more, thereby reducing gamma-ray noise. The Device for Indirect Capture Experiments on Radionuclides, or DICER, works by illuminating a sample with a beam of neutrons, then looking at what comes out the other side to see what neutrons disappeared from the beam because the sample absorbed them. In cases where both DANCE and DICER have measured the same sample, we have exquisitely detailed information. If only DICER can study it, our knowledge is not as complete, but we can often extract the highest impact information, even on highly radioactive isotopes, with much less theory-dependence than the ATLAS work.
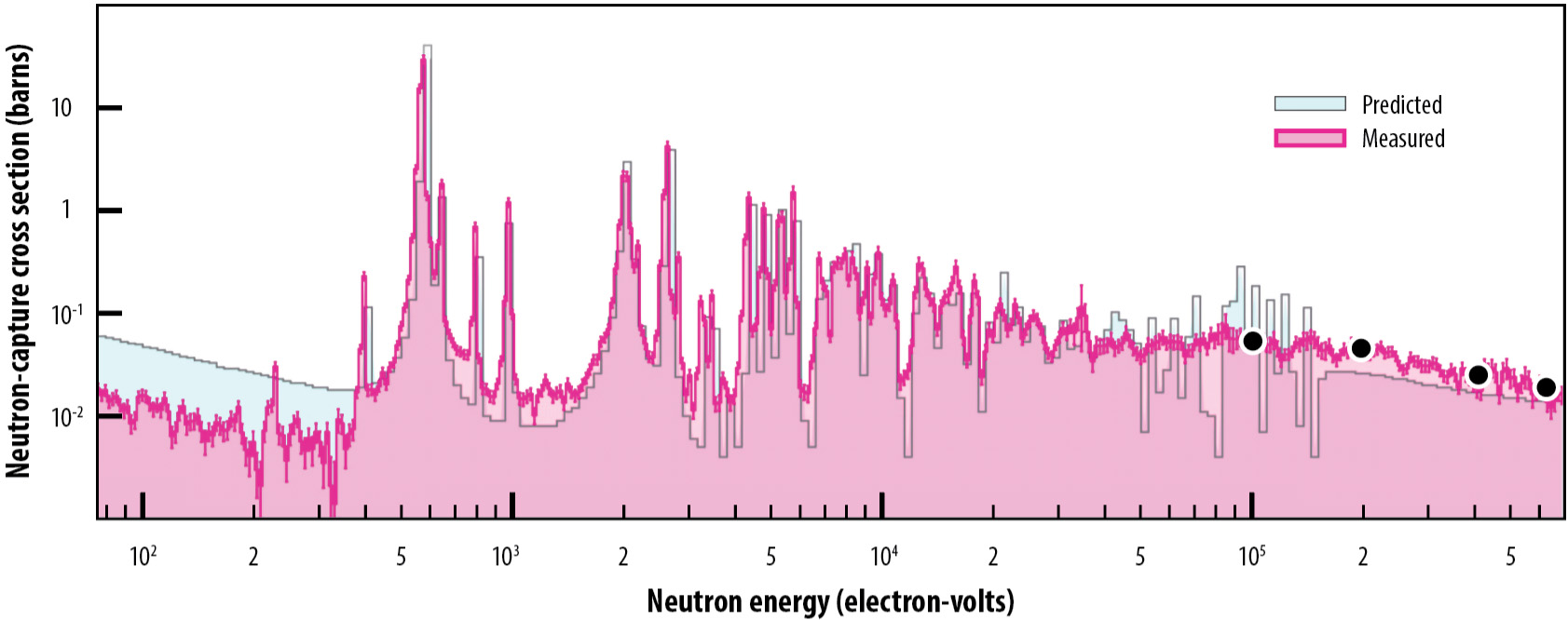
Between DANCE and DICER, through neutron capture and neutron transmission, we can measure the reaction rates of many important isotopes. But in some instances, the isotopes are so short lived that they can’t be made into a measurable sample. I’ve been developing, along with my colleagues Shea Mosby and René Reifarth, a revolutionary new idea for a completely different way to study neutron reactions at LANSCE. The measurements it makes won’t have the detail of DANCE and DICER data, but it will offer a way to directly measure reaction rates on very short-lived isotopes with no theory dependence—a tool we’ve never had before, and one that I believe we need. We call it MORD0R and it will turn the tables on how we measure neutron-induced reactions.
Neutron soup
The Multi-Orbit Ring Doing Zero-charge Reactions, or MORD0R, is an experiment that is still in the conceptual stage, but is very much about building a future capability at LANSCE.
In traditional neutron capture experiments, you have a beam of neutrons, you hit a sample with them, and then look for what reactions occurred. The problem is you might want to study something that is radioactive or has a short half-life and the beam-to-target paradigm just doesn’t work.
With MORD0R, instead of holding the heavy isotope sample still and bringing in a beam of neutrons, the neutrons hold still, in a sort of cloud called a standing field, and the heavy isotopes run through continuously. The neutrons are trapped in a moderator, more or less as a neutron gas, while the isotopic ions travel around a ring, passing through the neutron gas again and again, each time with a chance to interact with the neutrons. Imagine a car race on an oval track and along one length of the track there’s a sprinkler on, so that every time the cars pass through that length of track, they might get sprinkled. The cars are the nuclei, traveling around a ring, the sprinkler is the standing field of neutrons, and a drop of water on a car is a captured neutron.
When a nucleus picks up a neutron, becoming slightly heavier, the speed at which it races around the ring gets slower. With a well-designed ring, it is possible to measure how long each particle takes to make a circuit. When a particle suddenly appears with a slower circuit time, we know that a neutron capture has taken place.
MORD0R would allow us to actually measure nuclei that we have so far had to rely on theory to study.
MORD0R measurements will be direct reaction-rate measurements of the quantity of interest, unlike the convolution of measurement and theory that Apollo offered. MORD0R will answer the specific scientific question at hand, but it will never provide the rich information about the nucleus and measurement fidelity that DANCE and DICER offer. From those we get more than 1000 independent data points—often more than 5000—for each nucleus measured. From MORD0R, we expect to get 5–10 data points. But they will be the right 5–10 points, from the most relevant energies, to answer key questions for important fields—stockpile stewardship, nuclear astrophysics, nuclear energy, and basic nuclear science. MORD0R will mean almost any isotope can be measured directly, if needed.
The challenge with MORD0R is bringing together disparate technologies to operate in concert. We, as a scientific discipline, have created standing fields of neutrons. We’ve created rare-isotope beams. We’ve run ion beams in rings, both as protons here at LANSCE and as heavy ions elsewhere. We haven’t yet brought them together, and that is the research and development that is critical work to do today. Once we put all of those things together so they happen at once, we will have built MORD0R.
MORD0R will by no means replace DANCE and DICER. Those experiments will still provide the gold standard for truly understanding a nucleus and how it behaves most broadly. In fact, the spallation target they use—the piece of tungsten used to produce neutrons by bombarding it with protons—is being upgraded this year. MORD0R will complement and expand on DANCE and DICER, broadening our capability, and allowing us to actually measure the nuclei that we’ve so far had to rely purely on theory to study.
I came to Los Alamos because of the neutrons. I built my career here, largely hinged on neutrons. And the neutrons have plenty yet to offer, which we are still figuring out how to… figure out.