“Necessity is the mother of invention,” wrote Plato in Republic. Never have these words felt more apt than during a global crisis of historic proportion. Los Alamos National Laboratory was founded in such a time, and on a similar principle; the Lab’s original mission—to build the first atomic bomb—centered heavily on invention. The success of that mission set Los Alamos on a track of unwavering commitment to innovation and invention.
Today the world faces a different kind of crisis. The coronavirus pandemic and the myriad of challenges that have sprung from it have brought scientists and inventors clamoring to help. Through innovation in manufacturing, they are finding ways of increasing supplies, such as safety equipment and test kit components, to meet a ballooning demand, and they are looking for ways to help meet the demand with the supplies on hand. They are inventing new designs, testing new materials, and generating vital data so that new solutions can be brought to market as quickly as possible.
Innovators at the Laboratory are driven by professional commitment, personal circumstance, and global altruism. They are addressing all aspects of the problem, from preventing and detecting to treating and surviving infection by the novel human coronavirus, SARS-CoV-2. Here are some of the manufacturing projects that Laboratory scientists are working on to help with this pandemic and the next one.
Reducing the risk
“Make it like a dishwasher,” George said, “a dishwasher for N95s—I think it has to be that easy.”
It was April, 2020, and chemical engineers George Goff and Alex Marchi were brainstorming designs for a machine to decontaminate disposable N95 respirators using hydrogen peroxide vapor. Goff’s wife is a healthcare worker and there was serious concern in his house—and across the nation—that supplies of disposable personal protective equipment, or PPE, would run out. (N95s are colloquially called “masks,” but because they are engineered to form a seal on the wearer’s face and to prevent at least 95 percent of particles larger than 300 nanometers (nm, billionths of a meter) from passing through, N95s are not mere masks but true respirators.)
Hydrogen peroxide is an unstable, oxidizing species, meaning it easily strips electrons from other molecules. This can result in the formation of highly reactive free radicals, which can irreparably damage the virus’s envelope, proteins, and RNA genome.
Similar in concept to how large commercial units already operate throughout the country, Goff’s essential idea is to place many used N95s into a sealed container, saturate them with vaporized pathogen-killing hydrogen peroxide, then purge the toxic fumes and viola! Sterile mask, ready for reuse.
Smaller hospitals need smaller-scale solutions.
Whereas the large commercial units are great for the urban areas they serve, smaller cities and smaller hospitals need smaller-scale solutions. Unlike the urban units, which are shipping-container sized, take eight hours to sanitize a batch of a few thousand masks, and have trouble tracking which mask was whose, Goff and Marchi wanted their system to be much smaller, handle about a hundred masks at a time, and complete a cycle in just two hours. Furthermore, the commercial units rely on external subply channels for hydrogen peroxide—channels that fluctuate wildly in both cost and availability—while Marchi and Goff are building their system to generate its own.
The work draws on the considerable fuel-cell expertise that exists at the Lab. Fuel cells for cars, say, take oxygen (O2) and hydrogen (H2) and convert them into water (H2O). Hydrogen peroxide (H2O2) is an unwanted byproduct in fuel-cell chemistry, so it is a perennial challenge to keep its production to a minimum. Therefore, a lackluster fuel cell, one that makes too much H2O2, seemed like a good place to start to build an H2O2 generator.
“I called them up and said, ‘Give me your worst fuel cells—I mean the real stinkers,’” recalls Goff.
The team’s fuel cell-based H2O2 generator takes H2O and O2 (eventually perhaps plain air) and combines them into H2O2. As of this writing, it is producing H2O2 concentrations of about 7 percent (in water), which may seem low but the goal is just 10 percent, so it’s almost where it needs to be. The problem, suspects Laboratory fuel-cell scientist Rod Borup, may be decomposition. H2O2 decomposes easily—both light and metal will cause it to break down (that’s why it’s sold in dark plastic bottles). The team is searching for hidden causes of decomposition within their system and is confident that the 10 percent threshold is within reach.
Also from prior fuel-cell work at the Laboratory come excellent custom chemical sensors. Commercial H2O2 vapor sensors are expensive, short-lived, and reach their lower limit of detection exactly at the OSHA upper limit of one part per million, above which it is considered unsafe to breathe. Goff and Marchi wanted a cheaper, longer-lasting, more sensitive sensor and found it in the adaptation of Laboratory carbon dioxide sensors that had been previously developed for fuel cells.
“The sensors really are central,” explains Marchi. “You need to know that your N95 has really been decontaminated—that the chamber reached the necessary concentration and maintained it for the right amount of time—but equally important is the need to know, when you take it out, that you aren’t breathing in residual harmful vapor.”
The team is following Goff’s vision of making it like a dishwasher: It’s about the same size; the masks nest like bowls into pullout racks; and the user closes the door, pushes the button, and two hours later fresh masks can be distributed to their wearers.
This project is a collaboration that is bigger than just Marchi, Goff, and Borup; it brings together experts in fuel-cell chemistry, gas-sensor technology, 3D printing for prototype production, and fluid dynamics within the vaporization chamber. Although the panic over possible PPE shortages has relaxed a bit since April, the team believes it’s still worth bringing this project across the finish line. First of all, the pandemic isn’t over. Second, sooner or later it will happen again. Finally, and perhaps most poignantly, even in non-pandemic times, healthcare workers need reliable PPE, so having safe ways to reuse existing items helps curb waste while minimizing reliance on sudden influxes, whenever the need arises.
A lackluster fuel cell is a good place to start to build a hydrogen peroxide generator.
On the other side of the Lab another team is working on an entirely different way of sanitizing used N95s and other PPE for reuse.
“Our idea is to use ionizing radiation,” explains Los Alamos microbiologist Kumkum Ganguly. “It’s not a new idea; people have used gamma rays before. But we are uniquely situated here, and we are doing it differently.”
Ganguly and her Los Alamos colleagues Paul Peterson, Yongqiang Wang, David Seagraves, and James Hunter are taking advantage of the Laboratory facilities for nondestructive testing and evaluation, and for radiation protection services, to both eliminate infectious virus from PPE and study the effects, if any, the treatment might have on the materials. (Ganguly works with live coronavirus and collaborates on several other projects included elsewhere in this article to test inventions for virus-killing capacity.
First, they expose coronavirus-contaminated materials to either high-energy x-rays or gamma rays, then they compare the two methods to see which is best. Beyond comparing virus-killing capacity, the team is using computed tomography images and scanning electron microscope images to look at what structural changes, if any, the radiation causes in the PPE material. They are also determining changes in the materials’ electron-charge retention and filtration capacity to identify any functional changes caused by the radiation.
Both x-rays and gamma rays of sufficient intensity will quickly damage the virus’s genetic material beyond repair, rearranging chemical bonds within the molecule so that it becomes dysfunctional and even breaking the viral RNA into pieces. The scientists are doing a range of dose-determination processes, and Ganguly predicts that fairly low doses will be adequate to decontaminate PPE for reuse. This work reflects a unique collaboration between the Lab’s bioscience, materials science, and weapons science divisions, all working together on the same platform to fight the pandemic. The team is also collaborating with Microsoft Research for further development.
But it’s not just PPE that Laboratory scientists are decontaminating. Mechanical engineer Graham Arinder is working on a machine to clean the very air. Pre-pandemic, Arinder was working mainly on proton radiography and additive manufacturing projects, but when the pandemic hit, he and his colleagues began thinking of ways they could help.
“In an enclosed space, aerosols will build up over time, even if everyone is wearing a face mask. It’s not realistic for everyone to wear respirators all the time,” explains Arinder. “So, when someone sneezes or coughs in a conference room or shared office or lab, we want to be able to clean the air in that room.”
There are two ways to do this: filtration and sterilization. Filtration removes particles above a particular size threshold, including pollen, dust, and pathogens, but doesn’t kill them. Sterilization kills but doesn’t remove airborne pathogens like viruses, bacteria, or fungi; the particles remain in the air but they are no longer infectious. Filters have to be monitored and regularly replaced, and in the era of coronavirus they are considered hazardous waste, demanding special disposal.
“From an operational standpoint,” says Arinder, “sterilization makes more sense than filtration. This was an area of research before the pandemic, but now it’s been fast-tracked to get it usable.”
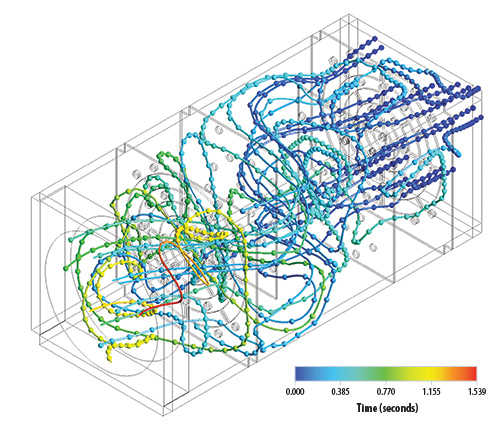
Arinder’s air sterilizer is basically an airtight box, about 20 cubic feet in volume, full of ultraviolet (UV) light bulbs (similar in appearance to the fluorescent tubes in office overhead lighting), with an air-handling system to move the air through. The air is drawn in, passed through a gauntlet of UV light where any pathogen’s genetic material is irreparably damaged, and blown back into the room once sterile.
The length of time that air spends inside the unit is a balancing act: Fast circulation produces better turnover but is less efficient at killing airborne viruses, while slower circulation kills the virus more effectively but leaves infectious virus lingering in the air for longer. Arinder believes the sweet spot is about 7–10 full air changes per hour. This is similar to the standard that hospitals use for filtration of air in patient areas.
The air sterilizer could be installed permanently or used on a temporary basis because it doesn’t require any building retrofitting or special ducting—it sits on casters and can be rolled into any room where it’s needed. The technology is also scalable to almost any room size. Furthermore, the cost of occasional light-bulb replacement is a fraction of the cost of regular filter replacement for air-filtration systems. Arinder is still fine-tuning both airflow and UV intensity; for this he is collaborating with scientists at Sandia National Laboratories and students at Texas A&M University, who are working on similar systems to decontaminate the air coming out of COVID-19 patients’ ventilators.
Arinder never thought he’d be inventing an air sterilizer, just as Ganguly hadn’t planned on irradiating PPE, and Goff and Marchi didn’t know they would be building a dishwasher for N95s. But in a global emergency like this, when decontamination suddenly matters like it has never mattered before, innovators across the Lab and around the world look at their skill sets and ask themselves, “How can I help?”
Changing the conversation
“Early on, the materials needs were changing almost continuously,” recalls Laboratory chemical engineer Matt Lee, who oversees several manufacturing efforts aimed at remedying shortfalls and shortcomings of single-use consumable supplies.
“Depending on the day, one might hear anything from ‘We need ventilator parts! We need face shields!’ to ‘Wait, never mind, now we need test swabs!’ Everyone was eager to help,” Lee continues. “We were spinning our wheels a bit; it was hard to make a clear plan that could have tangible impacts. But then we realized that instead of reacting to the news of the day, we should be anticipating the news of tomorrow.”
Filtration removes viruses but doesn’t kill them; sterilization kills them but doesn’t remove them.
One project Lee and others are working on is the development of a virucidal respirator: a reusable respirator that not only filters the air to keep pathogens out but actually kills pathogens that come into contact with it.
“Our goal is to make reuse a better option,” explains Lee’s collaborator, Laboratory electrical engineer Nina Weisse-Bernstein, who leads the project. “When a healthcare worker is faced with a shortage of PPE and has to make the choice of whether to reuse a mask or take a fresh one from an ever-dwindling supply, the use of virus-killing materials within the PPE itself makes reuse far less risky.”
N95 respirators, whether disposable or reusable, rely on fiber-based filters to prevent infectious viruses from passing through; fibers are layered randomly over one another until a certain level of occlusion is achieved. Weisse-Bernstein is developing a reusable respirator with a filter that not only traps pathogens but kills them because the filters are made of copper.
The natural ability of copper to kill pathogens on contact has been appreciated by the medically minded for nearly 7000 years; however, the exact mechanism by which it does this is still unclear. Perhaps copper ions disrupt the electrical potential across a microorganism’s membrane, or maybe they recruit damaging reactive-oxygen species, or they may interfere with the transcription and translation of the pathogen’s genetic material, or maybe the effect is a combination of these activities. In any event, it takes four hours on a copper surface for 95 percent of infectious coronavirus to be killed; by the end of a 12-hour shift, any virus that came into a nurse’s respirator filter during the first eight hours would almost certainly be dead.
Weisse-Bernstein’s team is using 3D printing to produce copper nanofoams. A prototype is 3D-printed from a mixture of copper and plastic polymer, then the polymer is baked away, leaving just the copper in a foam-like configuration.
Foam has different morphology than stacks of fibers, so a foam-based N95 will have a different airflow. The scientists are studying the airflow through various versions of copper foam to see how breathability is affected. With the 3D-printing method of manufacture, the number, size, and density of open pores can be tuned to achieve the best combination of filtration capacity and breathability. The variable that seems to matter the most in terms of breathability is the width of the copper material in between the pores, while filtration capacity is most affected by pore size.
The copper filter will attach to reusable N95 respirators. Such an antimicrobial option for N95s would mean filters don’t need to be changed nearly as often and would also drastically reduce the need for disposable N95s. Because it’s not coronavirus-specific, this technology would protect healthcare workers against other respiratory pathogens too. Though driven by present-day needs, it could truly be a paradigm shift for the future of respiratory PPE.
Another 3D-printing-based project at the Lab addresses shortages of consumables used for detection and treatment of infection. Testing and treatment involve many single-use items, from swabs and tubes to ventilator hoses and hose adapters; if any one of those supplies runs out, testing or treatment could be stymied. So, Lee and colleagues are exploring ways of making them faster, cheaper, or reusable.
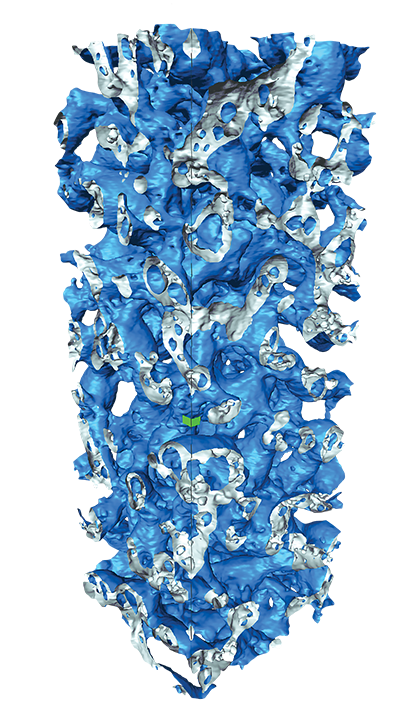
“The Laboratory is researching what could happen if a particular material supply chain is disrupted, and we’re looking at ways to address those problems,” explains Lee. “We aren’t manufacturing consumable products in large supply per se, but rather developing new manufacturing technologies and designs that are more robust to those disruptions.”
Laboratory scientists build prototypes for new concepts and do the science to prove the prototypes work. Once the proof-of-concept work is done, the Lab hands it off to partners in industry to bring the products to market.
The Laboratory’s 3D-printing capability is a key resource enabling rapid design and prototyping. Alex Marchi (of the N95 dishwasher project above) is a 3D-printing expert and is working with Lee to rapidly produce and test prototypes from new designs and new materials for single-use supplies. She is making things like plastic tubes for testing reagents, flow regulators for ventilators, sticks and packaging for nasal swabs, and more.
Marchi makes these items using stereolithography—a 3D-printing technology wherein plastic is laid down in liquid form, then UV light is used to solidify and bind the layers together to form a specific object. Marchi is exploring different kinds of plastic as well as different designs for making faster and cheaper consumables. If the item is to be reusable, then it also needs to be able to withstand decontamination methods, like heat, bleach, hydrogen peroxide, or ionizing radiation.
It’s pretty straightforward to imagine how some consumables, like swabs, tubes, and hoses, might be used in a clinical setting. But Los Alamos scientists are also developing other, very particular items whose utility can only be conceived by those in the trenches, the healthcare workers treating COVID-19 patients.
Edith Danielson is a hospital respiratory therapist who is married to Laboratory physicist Jeremy Danielson. In early 2020, Edith and her colleagues were warned to expect a shortage of ventilators, the life-support machines that keep unconscious patients breathing.
“We sat down to talk about how to prepare for the coming shortage,” recalls Edith, “and we decided that in addition to trying to meet the demand for more ventilators, we wanted to look for ways to reduce the demand as well.”
Ventilators aren’t the only machines that help people breathe—positive airway pressure (PAP) machines are prescribed to thousands of people each year to help treat sleep apnea. Edith knew that PAP machines are much more abundant than ventilators, and it seemed to her like a promising option if and when the shortage arrived.
The challenge with using PAP machines to help COVID-19 patients breathe is that these machines have exhale valves as well as emergency anti-asphyxiation vents that aren’t filtered, so the machine and the entire room would quickly fill with exhaled coronavirus. The Danielsons, along with Edith’s manager at the hospital, Daniel “Scotty” Sylva, teamed up with Lee and Los Alamos electrical engineer Jeremy Payton, and the group began discussing ways of retrofitting a filter onto a PAP machine. Viral filters for breathing tubes exist, but the diameter for the filters doesn’t match the diameter of PAP machines’ breathing tubes, so it was a matter of making an adapter to fit them together. Laboratory research technologist Ruben Manzanares was brought in to help turn rough ideas into actual parts that could be tested.
It takes 4 hours on a copper surface for 95 percent of coronavirus to be killed.
As the pandemic progressed, the team began seeing more reasons to choose PAP over ventilation. First, a patient needs to be sedated for ventilation and can’t be taken on and off as needed. Second, the needs of COVID-19 patients, in terms of air pressure and flow, tend to fluctuate dramatically, and PAP machines are more nimble than ventilators for these kinds of adjustments. Third, it was becoming apparent that ventilators weren’t necessarily improving patients’ outcomes. Ventilators aren’t meant to be used for more than a few days, but COVID-19 patients can be kept on for up to 60 days, during which time their lungs may be damaged not only by the infection but by the ventilator itself. So the team started thinking about PAPs not as ventilator stand-ins, but as ventilator preventatives—a way to circumvent the vents.
The invention is an adapter that needed to be simple, easy to use, and made from nontoxic material that can be sterilized (an early version melted in the autoclave). The team tried different designs and different materials and finally arrived at something that worked.
“The adapter is a relatively simple part,” says Jeremy Danielson, “but it’s a part that the medical community asked for, and we are happy to supply a design.”
And the team didn’t just make one adapter, they made two.
Another problem that Edith and Scotty saw while treating COVID-19 patients arose during intubation. Inserting a breathing tube into a patient’s trachea is a potentially messy procedure, made dangerous by the coronavirus. COVID-19 patients have to be intubated with their upper bodies inside a clear plastic glovebox to protect the medical staff, but it makes a difficult procedure even harder. Edith wanted something to suck the air away from a patient’s face, thereby creating a small area of negative air pressure, so that a medical worker could quickly intubate the patient without inhaling any of the patient’s exhaled air.
This type of machine exists; it’s called a smoke evacuation unit and is used during surgery to evacuate smoke after cauterization, for example.
“If you hook a face mask to a smoke sucker, to suck exhalations away,” Edith explains, “the suction is too strong, and the mask sucks down onto the patient’s face, blocking access to the airway.”
The adapter the team made to turn a smoke sucker into a virus sucker is a plastic bridge that supports a face mask—the clear plastic kind used in hospitals to administer oxygen—by holding the face mask close to the patient’s mouth, while keeping it out of the way. The team designed and fabricated two versions: one for emergency use and one that takes into account patient-comfort considerations for longer-term use.
This setup would be particularly good for hospitals with limited resources that lack negative-pressure rooms. Even small hospitals have built-in vacuum lines, which, with the addition of in-line virus filters, could safely be used to similar effect as a smoke-evacuation unit. The ability to create a negative-pressure zone would also be useful for other respiratory pathogens, like influenza or tuberculosis, or during the administration of toxic medication.
“This is one of the most fulfilling projects I’ve worked on,” says Payton, who, before the pandemic hit was working on subcritical nuclear weapons experiments at the Nevada National Security Site. “When Edith brought these ideas to us, we started looking at them right away. It felt good to be helping the hospital workers directly.”
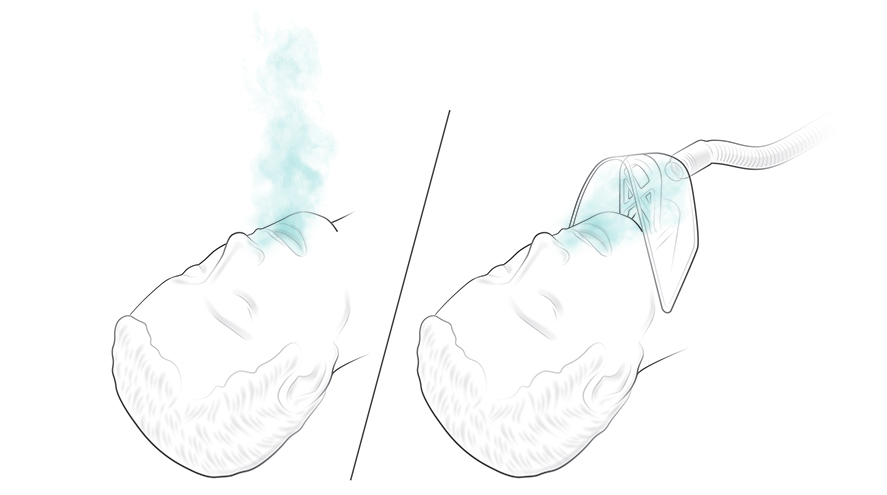
The team has provided prototypes of both inventions to a local hospital for evaluation within its laboratories, where the data needed for Food and Drug Administration (FDA) approval and industry partnerships will be collected.
Delivering the data
For a new device, material, or design to receive FDA approval, it needs to come with a litany of performance data. For some pandemic-related inventions, the design is done but the data are lacking. Here too, Laboratory scientists are helping by designing and conducting experiments to provide the missing numbers.
For example, early in the pandemic there was a lot of discussion about whether laypeople should wear masks, and if so, what kind and under what circumstances.
“Do masks actually make sense?” asks Laboratory physicist Michael Ham. “It helps to have data to inform those types of decisions, so we did the experiments.”
Ham and colleague Yong Tao use a cough machine, designed by Laboratory mechanical engineer and airflow expert Murray Moore, to evaluate how well various types of material might help contain the expelled virus. What kind of homemade mask would have the best filtration capacity: Bandana? T-shirt? Fancy bedsheets?
The cough machine sprays droplets of fluorescent liquid onto a homemade mask with a piece of filter paper behind it to catch whatever passes through. Then the proportion of droplets that are caught on the mask is compared to the proportion on the filter paper. The smaller the droplet, obviously, the more likely it is to go through, but also, and perhaps less obviously, the less likely it is to be infectious.
A SARS-CoV-2 particle is about 0.1 micron in diameter (a micron, or µm, is a millionth of a meter), and N95 respirators are effective at filtering down to about 0.01 µm. Speaking produces droplets of about 1 µm, and coughing produces droplets of about 200 µm. It has been estimated that a 10-µm droplet has a 37 percent chance of containing a single virus particle, so while coughing produces droplets large enough to contain at least one virus, speaking usually doesn’t. The infectious dose, or the number of SARS-CoV-2 particles it takes to successfully infect a person, is difficult to determine, but scientists suspect it might be as low as a few hundred. The team concluded that homemade masks are actually quite effective for the most relevant droplet sizes.
“There’s an argument I’ve heard that a cloth mask keeps SARS-CoV-2 out as well as a chain-link fence would keep a mosquito out,” Ham explains. “But that’s a false analogy in most instances. You’re looking to catch droplets, not single viruses, so it would be more like using a chain-link fence to keep out a trash bag full of mosquitos.”
The scientists found that if two people are wearing homemade masks and standing in pre-pandemic proximity, about 50 percent of respiratory droplets produced by Person 1 will be caught by Person 1’s mask, while 50 percent of the droplets that passed through will be caught by Person 2’s mask. So only 25 percent of the droplets leaving Person 1’s airway might enter Person 2’s airway, and both masks participate in reducing the risk for both people. This factor-of-four reduction can be further reduced if the two people keep a larger distance between themselves than they would have done during pre-pandemic times, so that most of the droplets leaving Person 1 will fall to the ground before reaching Person 2.
The exception to this is healthcare workers. Medical staff who are spending prolonged periods of time in confined spaces with confirmed, highly infectious patients will receive inadequate protection from homemade masks. Single SARS-CoV-2 particles can linger in the air and accumulate to a dangerous concentration in such a circumstance. So medical workers absolutely need N95s to do their jobs safely, but for the rest of us, homemade masks will do for picking out produce at the grocery store.
Homemade masks are surprisingly effective for relevantly sized droplets.
In addition to the cough-machine tests, the team did a series of particle transport tests using a wind tunnel that Moore had previously built at the Lab for air-quality testing.
Team member Rebecca Williams, who is an industrial hygienist and the Laboratory’s PPE subject-matter expert, explains, “As forward velocity changes by talking or breathing, the filtering capabilities of the masks can change as well. Expelled air contains moisture, and as the day goes on, the mask can accumulate and retain that moisture, causing it to be weighed down, which can change how the mask fits, and thus its filtering efficiency.”
The scientists studied forward air velocity and particle permeation through various materials to determine how novel materials would perform for all-day wear. The team also evaluated which materials would best impede forward air velocity, limiting one person’s breath from reaching another person’s breathing zone. For example, they determined that air exhaled while speaking without a mask travels fast enough to register on an anemometer at 10 feet, but only 2 feet with a mask. They also found that cloth masks remain effective for up to six hours in the wind tunnel.
Using a pseudo-saliva made from water, glycerin, and table salt, the team did evaporation tests. They found, for example, that a 5-µm droplet traveling at 1.5 meters per second will fully evaporate during its 20-foot flight from generator to detector. This matters because evaporation quickly reduces the size of droplets, correspondingly reducing their filterability.
So outside of a healthcare setting, virus-containing droplets produced from speaking and breathing are very likely to be caught on the speaker’s mask. If a droplet does go through, its velocity has been impeded so it won’t travel as far, but if it does reach another person, and that person is also wearing a mask, their mask provides another chance to catch the droplet.
“We found that masks do work to stop the spread,” says Ham. “I think it would help consumers understand this if store-bought masks were to have ratings that tell their filtering capacity and breathability. Our data ought to help that happen.”
Another project that is helping provide necessary data is a ventilator testbed established by Laboratory engineer Todd Jankowski. Earlier this year, when the country was expecting to run out of ventilators, inventors began designing low-cost, easy-to-source medical respiratory devices, casually dubbed “DIY ventilators.” Not actually DIY (“do it yourself”), in the sense that one can’t put oneself on a ventilator—it requires full sedation and a medical team—the DIY ventilator designs use parts found at hardware stores and are intended for hospitals that are overwhelmed by COVID-19 patients in need of breathing assistance.
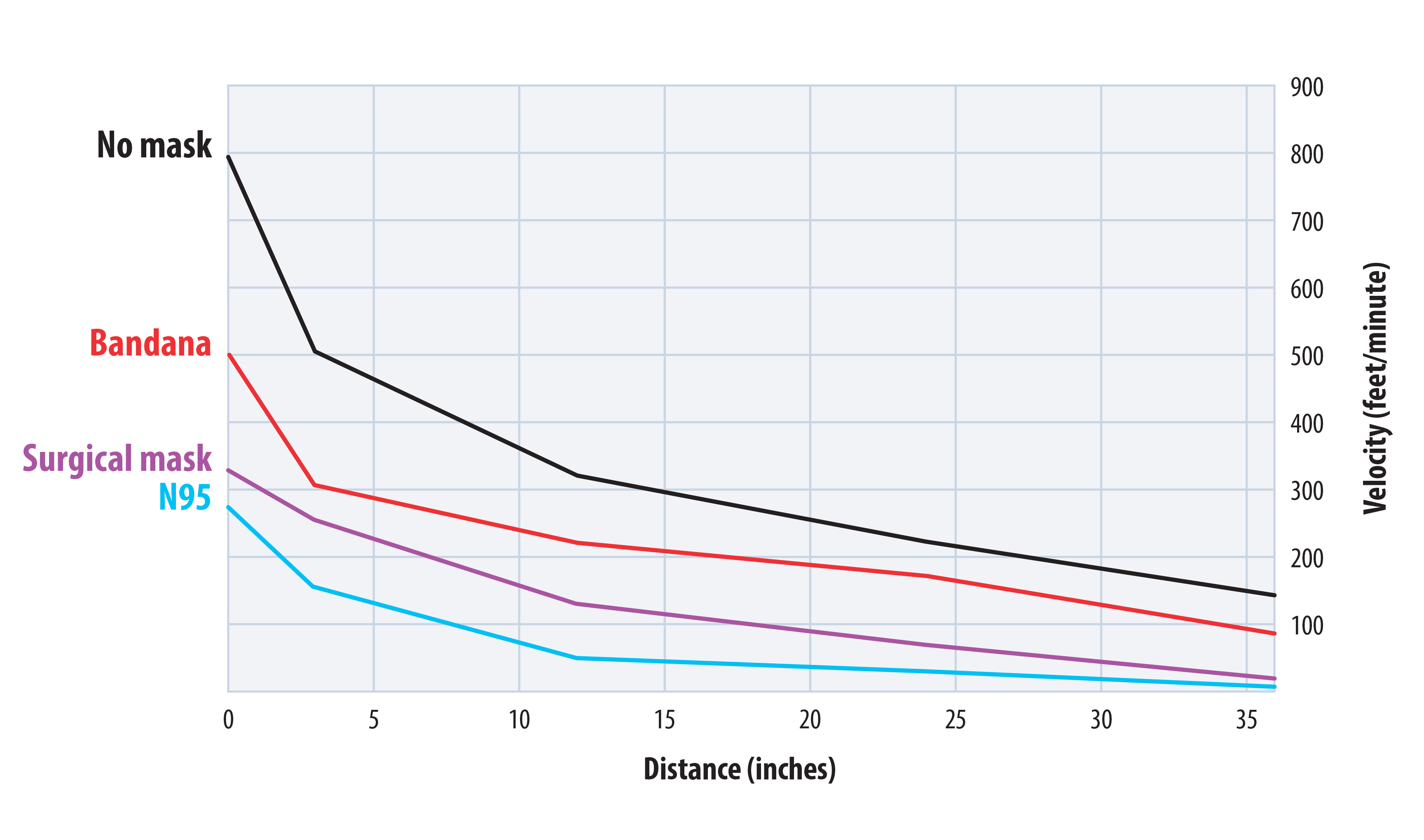
Jankowski didn’t design a DIY ventilator; he designed a rig that can test how well a DIY ventilator performs. The ventilator testbed and its personnel do three things for a DIY design: they test the device as designed, refine the design, and produce detailed, easy-to-use assembly instructions. First, Jankowski and his team build the DIY ventilator according to the designer’s instructions, then they outfit it with pressure, flow, and oxygen sensors and collect performance data. While building and operating, the engineers and technicians on the team look for possible design improvements and suggest solutions if problems are encountered. Once the design is final, they write and illustrate detailed, user-friendly assembly instructions. Armed with the test data and the assembly instructions, the inventor can now apply for a patent and FDA approval.
Along similar lines to the ventilator testbed comes another Laboratory project that is looking at a specific type of commercial ventilator to understand how it might be used for COVID-19 patients. Intrapulmonary Percussive Ventilators (IPVs) pulse fine aerosols into a patient’s lungs to help break up and move mucus. IPVs are typically used to treat patients with severe asthma or premature infants whose lungs aren’t functioning properly.
“We wanted to study the science and engineering of the IPV because it’s never been used before for COVID-19,” says Laboratory engineer John Bernardin, who leads the project. “What we’re trying to study is how the aerosol generated from an IPV affects mucus that resides in an infected lung. Does it help clear a path for air to get in?”
The experimental setup is a 3D-printed model of the first three branches of the human bronchotracheal system (there are 23 branches in all). The model is equipped with various sensors to determine how air moves down lung passages and how it changes in terms of concentration and droplet-size distribution. An artificial lung is attached to the model, and as it breathes in and out, the researchers can see how the aerosol changes. There are also optical sensors that can watch if and how a patch of lab-grade mucus, applied to the inside of the 3D-printed model, is affected by the IPV.
Knowing how to best use our tools is going to help determine best practices for treating COVID-19.
The data from these and other experiments, as well as data produced by computational fluid dynamics modeling, are used to train a machine-learning algorithm that can predict the effect of various IPV treatment settings. Knowing how to best use the tools that we already have, as well as inventing new tools, is going to help the medical community determine best practices for treating COVID-19.
Readying the resources
Collaboration lies at the heart of scientific endeavor, especially now when the world is united in the common cause of ending the coronavirus pandemic while saving as many lives as possible. Many of the projects described here are larger than Los Alamos; they are national multi-institution collaborations established to solve pandemic problems quickly and permanently.
Lab scientists are working to ameliorate supply shortages by finding new ways to produce things and inventing altogether new things. From gas sensors to ventilator adapters to new kinds of PPE, Los Alamos designs and data will help the Laboratory’s partners in industry bring vital new technologies to market.
It takes time, effort, and expertise to get new technologies going. Racing against the clock and achieving success under the gun is something the Laboratory has specialized in ever since its first mission. Now, as then, when the stakes are highest, Los Alamos scientists are answering the call. LDRD
Testing the Tools
Los alamos microbiologist Kumkum Ganguly works with scientists to determine if and how well their machine or material kills coronaviruses. For items like Arinder’s air sterilizer or Weisse-Bernstein’s copper filter, it is crucial that the virus truly be eliminated as expected. But for other things, like Marchi’s 3D-printed test components, it’s vital that the material not kill the virus, lest the test report a false negative.
Los Alamos is not equipped for work with live SARS-CoV-2, so Ganguly and her team, Samantha Adikari and Seychelles Voit, use less pathogenic surrogates: two human coronaviruses, termed HCoV-229E and HCoV-OC43, both known causes of the common cold, that are structurally similar to SARS-CoV-2.
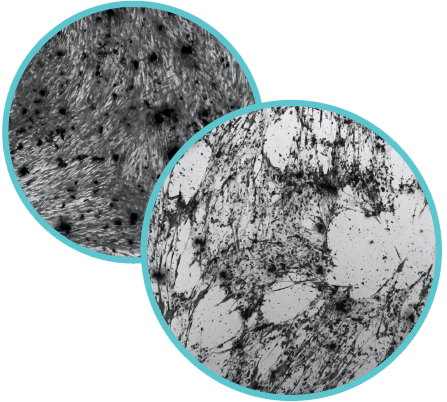
monolayer with all of the cells a relatively uniform size.
Right: Infection is apparent when holes appear in the monolayer.
The holes form when infected cells die, losing adhesion from their
neighbors and bursting to release thousands of new viruses
The scientists culture human lung cells in plastic dishes containing a specialized liquid growth medium. When a coronavirus infects a cell, the cell becomes a virus-making factory, and when it becomes too full of virus, the cell bursts and releases thousands of new virus particles into the surrounding growth medium. The team uses this virus-containing medium to determine different items’ virus-killing capacity. After coating an item, say a small piece of novel polymer, in a known quantity of virus and incubating for a specific amount of time, the item is washed with fresh medium that will then be given to fresh, uninfected cells. If the new cells become infected, they will begin to die after a few days and the scientists know that either the item itself or the incubation time was insufficient to kill all of the virus.
Ganguly’s team also does reciprocal tests, called biocompatibility tests, which determine if coming into contact with the virus affects the material and, if necessary, how the material stands up to common sterilization methods. And because the materials have to be effective against any pathogen that a healthcare worker or patient might encounter in a hospital setting, the team does bacteria-killing and bacteria-growing tests as well.
Finally, once a new invention passes muster in Ganguly’s lab, it still has to be proven against actual SARS-CoV-2. For this Ganguly will collaborate with scientists at other institutions with special labs designed for safe handling of this dangerous virus.