Astrophysics is a land of mysteries. Some new discovery is made—a source that’s brighter than it should be, or a celestial event that should not have occurred—and astrophysicists like me set out to explain how it came about. It is usually our job to construct a coherent story for what is going on in our universe, explain the unexplained, and resolve the mysteries, all without a single in-situ measurement. Other times it is our job to look at the universe with fresh eyes, and when we do that, we identify new mysteries.
Case in point: Just last year, a new kind of observatory made an unanticipated discovery. Instead of measuring visible light with lenses and mirrors, or measuring radio waves with a big metal dish, the LIGO observatory measures gravitational waves—incredibly faint ripples in the fabric of spacetime—with exquisitely sensitive laser-based instrumentation in kilometers-long ultrahigh-vacuum tunnels. These gravitational waves are so weak that the only ones we can hope to see are created by extraordinary gravitational events, such as two black holes colliding. This happens from time to time. One such collision witnessed by LIGO last year involved the merger of two black holes with masses estimated at 66 and 85 times that of the sun. According to well-established theory, however, the process by which black holes form should max out around 50 solar masses. So where did these two come from?
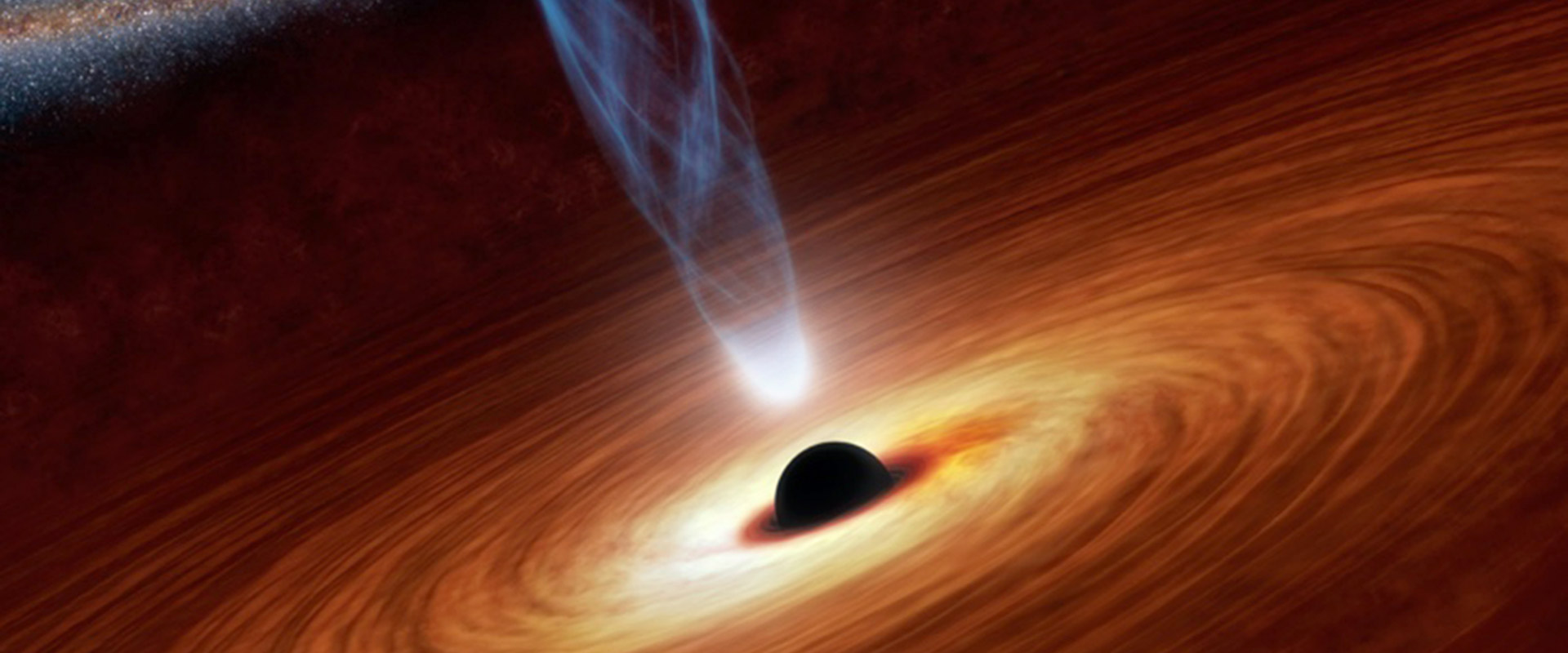
Or consider another detection at the IceCube Neutrino Observatory, a collection of detectors spanning a cubic kilometer embedded inside ice beneath the South Pole. IceCube watches for a faint, telltale flash of visible light that can, on rare occasion, result from a neutrino particle passing through the earth. In the past eight years, the observatory has documented numerous neutrinos with energies ranging up to about a billion times greater than the most energetic neutrinos ever seen before, which themselves came from a massive supernova. So what’s producing these wildly energetic neutrinos? What celestial power source could dwarf a supernova by a factor of a billion?
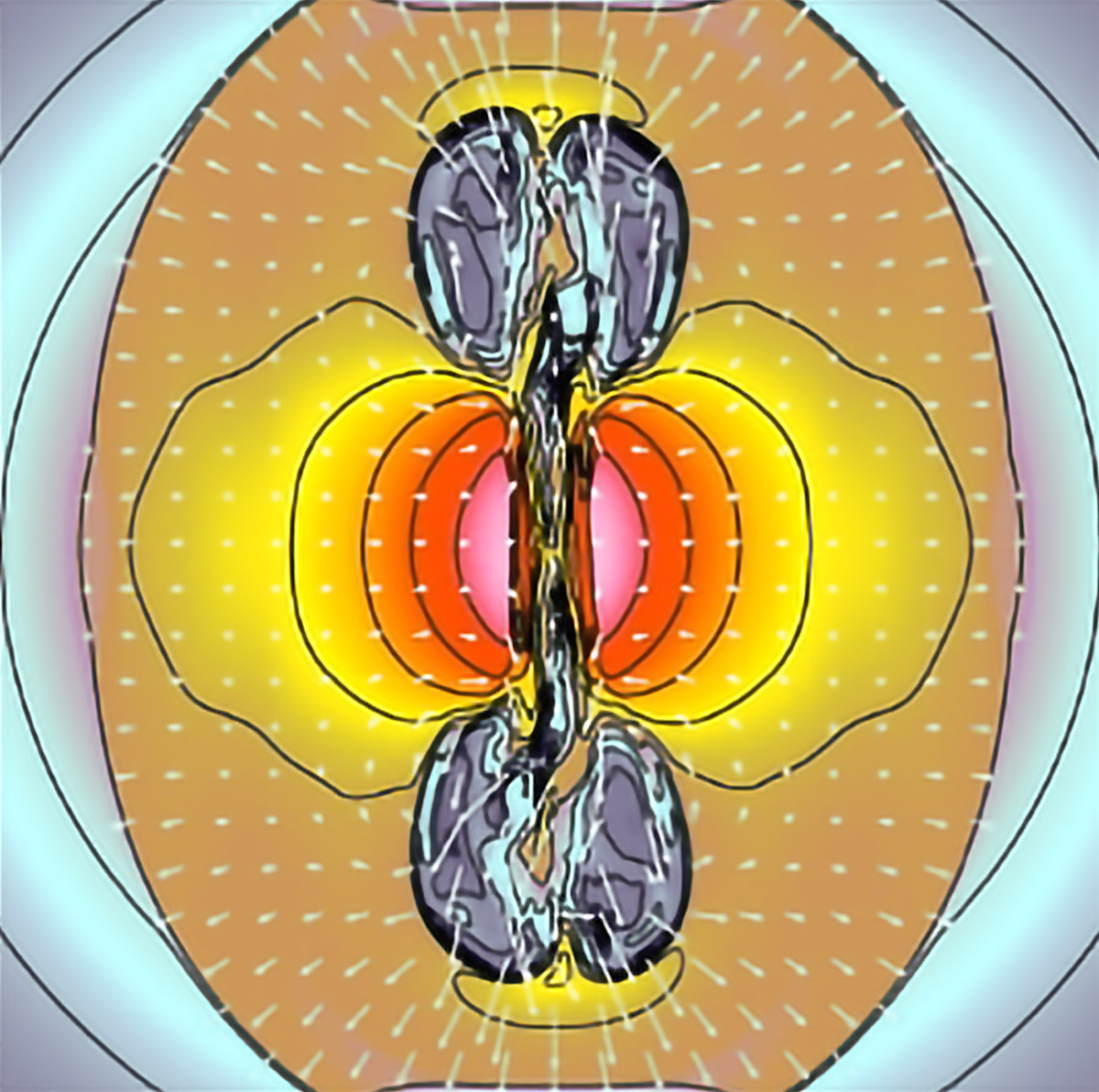
You know you’re in a fascinating line of work when progress means not only resolving mysteries like these but also creating them with some new kind of observation. Personally, I tend toward the former. I try to figure out how things work and resolve the mysteries that new observatories identify (and ideally make some predictions along the way). But nowadays, astrophysical mysteries are rarely resolved by a clean, straightforward idea that can be expressed with a single, textbook-style equation. Today’s mysteries tend to involve a great deal of complexity and require interdisciplinary approaches. I am fortunate to have developed expertise in complex, magnetized plasma systems, based on advances in theory, numerical simulation, and laboratory experiments. It has frequently meant that I can see my way to a possible solution to some high-energy mystery—including to the two I just mentioned. But we’ll come back to those after a little background.
Underappreciated magnetism
Relatively early in my 26-year career (so far) at Los Alamos, I had the privilege of working with a giant in the fields of astrophysics and plasma physics, Stirling Colgate. Stirling was part of what I think of as an earlier generation of scientists with the heart to tackle big, fascinating problems in science and do it without worrying about funding. It took guts, foresight, and an appreciation of the big picture to make a career doing that kind of work—a strong “let’s see where this takes us” spirit. (To be fair, he also worked prolifically on practical problems, such as thermonuclear weapons, satellite-based detection of nuclear detonations, and other innovative fusion research.)
So I try to maintain this entrepreneurial spirit, exploring the frontiers wherever they may lie—doing my part to keep Los Alamos nimble and its research pioneering. One such line of research that I have long pursued centers on a class of objects called supermassive black holes. They live at the centers of large galaxies, including ours, and, despite being as massive as millions or billions of suns, are only a fraction of the size of our solar system.
If one were to ask what are the ultimate hidden engines that drive most of the high-energy phenomena throughout the cosmos, supermassive black holes rank at the top. How can such comparatively tiny objects (tiny in size but huge in mass) be so important? Back in the late 90s, we developed a perspective on supermassive black holes and the universe that was not widely accepted at the time but has since come to be better supported: that magnetized jets are responsible for drawing energy from supermassive black holes and distributing it around intergalactic space—the sprawling space between galaxies—where it exists as the lion’s share of all energy present. (A plasma is a gas that is hot enough that electrons have been stripped off of their atoms, resulting in the particles that make up the gas being electrically charged—ions and electrons—unlike the neutral atoms that make up a lower-temperature gas, such as air. By virtue of being charged particles, plasmas interact strongly with magnetic fields.)
Supermassive black holes are pretty quiet in the universe today. But earlier in the history of the universe, they were screaming. There was an ample supply of gas surrounding a supermassive black hole, and as that gas was drawn in, it would speed up, with faster and faster particles colliding to generate a tremendous amount of heat. Meanwhile, these particles start orbiting around the black hole and settle into a flattened geometry called an accretion disk (their last resort to save themselves from black holes). There are two eventual outcomes. First, the accretion disk is heated dramatically, producing an extraordinarily bright glow—a glow we often refer to as a “quasar” (quasi-star). Second, the magnetic fields are reconfigured by the black hole and accretion disk in such a way that these fields store the black hole’s energy and blast away as jets (with some of the disk material mixed in) over the poles of the black hole. These jets, billions or trillions of times longer than the diameter of the black hole itself, act as intergalactic conveyor belts, sharing the black hole’s energy across the colossal scales of intergalactic space.
The touch of supermassive black holes can be felt absolutely everywhere, including here on Earth.
There’s something I find delightful in all this. Supermassive black holes are extraordinary power sources. The gravitational energy released by their formation and growth (as they consume matter falling in) is enormous. But together with being extraordinarily powerful, they are extraordinarily compact. One might naively expect that their impacts should only be felt in their immediate vicinities. Yet through the rich and complex dynamics of magnetically active plasmas, an amazing, self-organized structure emerges, namely, an astrophysical jet. As a result, the touch of supermassive black holes can be felt absolutely everywhere, including here on Earth.
We showed that over time, even after the supermassive black hole has consumed all the available gas and therefore gone quiet, the residual magnetic energy from the former jets would continue to spread throughout intergalactic space, filling up the volume in between massive galaxies. Quite interestingly, these jets are also believed to produce high-energy particles that zoom every which way across the universe and periodically streak through the earth’s atmosphere, raining down on us harmlessly (thanks to the earth’s precious atmosphere and magnetic fields) in the form of ultrahigh-energy cosmic rays.
A jet all the way
Like much of my research over the past 25 years, learning to understand supermassive black hole jets has relied upon detailed studies of turbulent magnetohydrodynamics (MHD) in the relativistic regime (in which particles are so energetic as to move at nearly the speed of light). This is exceptionally complicated, but it’s something of an historical specialty here at Los Alamos, since aspects of it are necessary in the study of the dynamics of fusion and nuclear detonations. (Manhattan Project-era giants, such as Fermi and Rosenbluth, were founding fathers of this field.) We have supercomputer codes designed to simulate the MHD and kinetic action of plasmas, and my colleagues and I have adapted and expanded these for astrophysical settings. Our computations and supercomputer simulations have shown that complex magnetic effects often dominate the dynamics of high-energy astrophysical systems.
There are two main dumpsites for black hole energy along a jet, each of which engenders exotic phenomena. My colleagues and I determined that large galaxy clusters—groupings of thousands of galaxies all orbiting around each other—are the primary dumpsites for most of the energy from supermassive black holes. About twenty years ago, we began conducting extensive MHD studies on the structure of jets, and it turns out that different magnetic field components conspire to maintain a tight, narrow helix structure (much like a slinky) over a long distance as the jet pushes through the interstellar gas within its host galaxy. A shock wave helps to harden the leading edge of the jet, preventing it from splaying out until the interstellar gas thins to the point where the pressure support is mostly gone. Then the jet spreads out into broad lobes, seeding the intra-cluster medium and the intergalactic medium with magnetism.
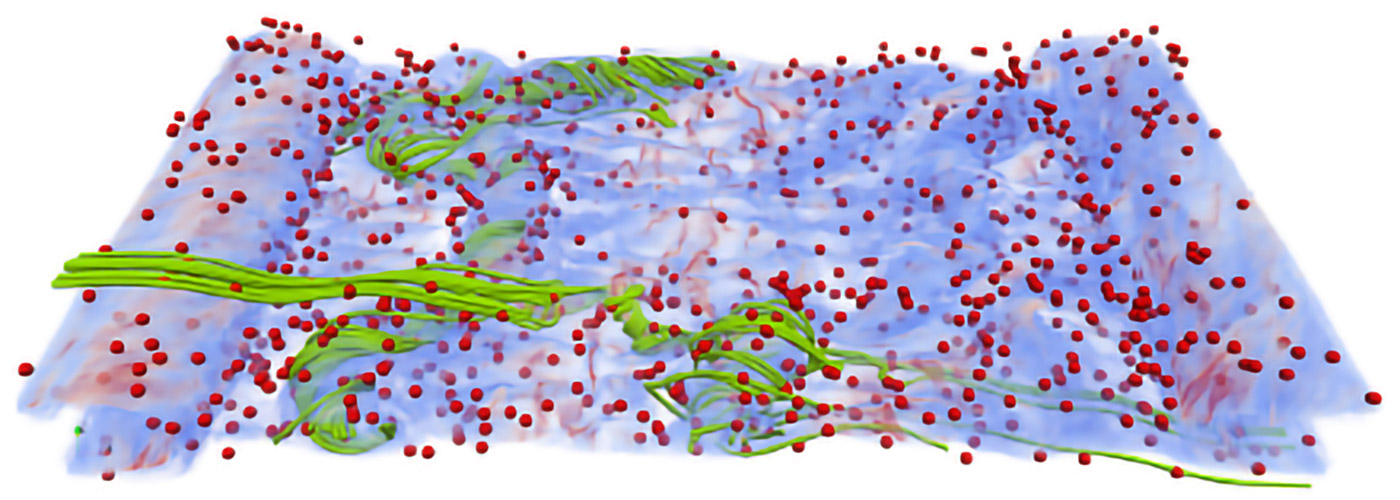
One key signature we demonstrated is how the turbulent (erratic, clumpy) gas motion inside the clusters can sustain and amplify the magnetic fields. We further showed that the dissipation of this magnetic energy helps maintain the cluster’s thermal energy, thereby resolving a longstanding mystery about how hot x-ray-emitting gas within galaxy clusters continues to glow long after it should have cooled: it is continuously recharged by turbulence-enhanced magnetic energy, seeded by supermassive black hole jets.
However, jets are imperfect conveyor belts for carrying black hole energy to great distances. The other dumpsite—and in some ways the more exotic one—is actually within the first one-millionth of the conveyor belt’s length. Here, dazzling flares of intense radiation, high-energy particles, and neutrinos are produced suddenly. Magnetic field lines within the jet are bent to the point of kinking, forcing the lines to reorganize, reconnect, and convert magnetic energy into motion, as well as greatly accelerate charged particles. In fact, when the Trinity supercomputer was first brought to Los Alamos in 2017, we were fortunate to be part of a team that took it for a test drive. With it, we demonstrated that particles in magnetized jets can indeed be accelerated and obtain a distinctive velocity distribution—not the usual thermal distribution associated with glowing objects like stars—which helps to explain the energy spectrum that has long been observed in jet-driven astrophysical systems (which also include x-ray-emitting binary star systems and gamma-ray bursts).
One telltale sign of magnetically regulated processes is the polarization of emissions made by energetic particles in the magnetic field. Gyration of these particles around magnetic fields causes the emission in the direction perpendicular to the magnetic field to be different from that in the parallel direction. To test our models, we were compelled to integrate three distinct components together: how relativistic jets emanate from supermassive black holes, how the particles get accelerated in such jets, and what radiation they produce. We got quite excited when we found that large changes in polarization behavior (which are most naturally explained in strongly magnetized conditions) could be generated during flares, a puzzling fact that had been reported by observations.
There are two main dumpsites for black hole energy along a jet, each of which engenders exotic phenomena.
Speaking of resolving mysteries, remember I mentioned that a current mystery is the origin of the wildly energetic neutrino particles detected by the IceCube observatory at the South Pole? Well: a 2017 neutrino detection happened to be coincident with radio and gamma-ray signals seen by other observatories, corresponding to an energetic flare from a “blazar”—a distant supermassive black hole jet that happens to be pointing toward us (i.e., a quasar that’s oriented just-so). This suggested that particle acceleration within the jet might be responsible for generating the mysterious neutrinos, probably via a known mechanism by which high-energy protons immersed in an electromagnetic glow (radio waves, visible light, etc.) can undergo particle-physics reactions that result in neutrinos.
My colleagues and I studied the event and explained how the magnetic forces and plasma dynamics within the jet could lead to sufficiently energetic neutrinos and even how the fine details of the necessary proton acceleration within the jets could be resolved with upcoming observational studies. This research is particularly exciting because it capitalizes on “multi-messenger” observations: neutrino telescopes, radio telescopes, x-ray and gamma-ray telescopes, all coordinating to converge on a single, coherent explanation of what is really going on in ultra-energetic systems.
That brings me back to the latest messenger in the modern era of multi-messenger astronomy: gravitational waves. When LIGO recently published a new discovery about the merger of two stellar-mass black holes (each with the mass of a large star, not millions or billions of stars’ worth like the supermassive black holes I’ve been talking about), and it sparked the mystery of how these black holes got to be bigger than the 50-solar-mass limit their formation mechanism should have imposed, I got very excited. My studies of supermassive black hole disks had previously led me to examine planet-forming disks around young stars and the conditions that allow planet embryos to survive and migrate within a disk, despite the drag the disk matter could impose [see “Wandering Worlds” in the March 2011 issue of 1663]. I realized that the same conditions could well be present in the accretion disk around a supermassive black hole.
The idea is that stars, even binary stars—and even dead binary stars that have collapsed into black holes—could have formed, lived, and died within the supermassive black hole’s accretion disk. And the binary black hole system LIGO detected has approximately the same mass ratio relative to the supermassive black hole it orbits as that of the earth relative to the sun: an intriguing commonality for these two wildly different systems. Thus, the LIGO system, unlike black-hole binaries people normally think of, could live inside a dense disk of material. So even if its black holes were born with less than 50 solar masses as theory demands, they’ve had plenty of nearby disk material to eat and have thus gained weight. Meanwhile, in a new publication this year, my colleagues and I were able to demonstrate that a narrow subset of binary black holes will indeed be driven to merge within an accretion disk (while the rest will tend to spin apart). It now seems to me exceedingly likely that this is the answer to the LIGO mystery. Even more interesting, the distinctive prediction of this scenario is that such mergers, due to the surrounding disk material present, will involve their own disks and jets and therefore emit concurrent, observable electromagnetic signals (in addition to gravitational waves), while models of isolated black-hole binaries said no such electromagnetic signatures should exist.
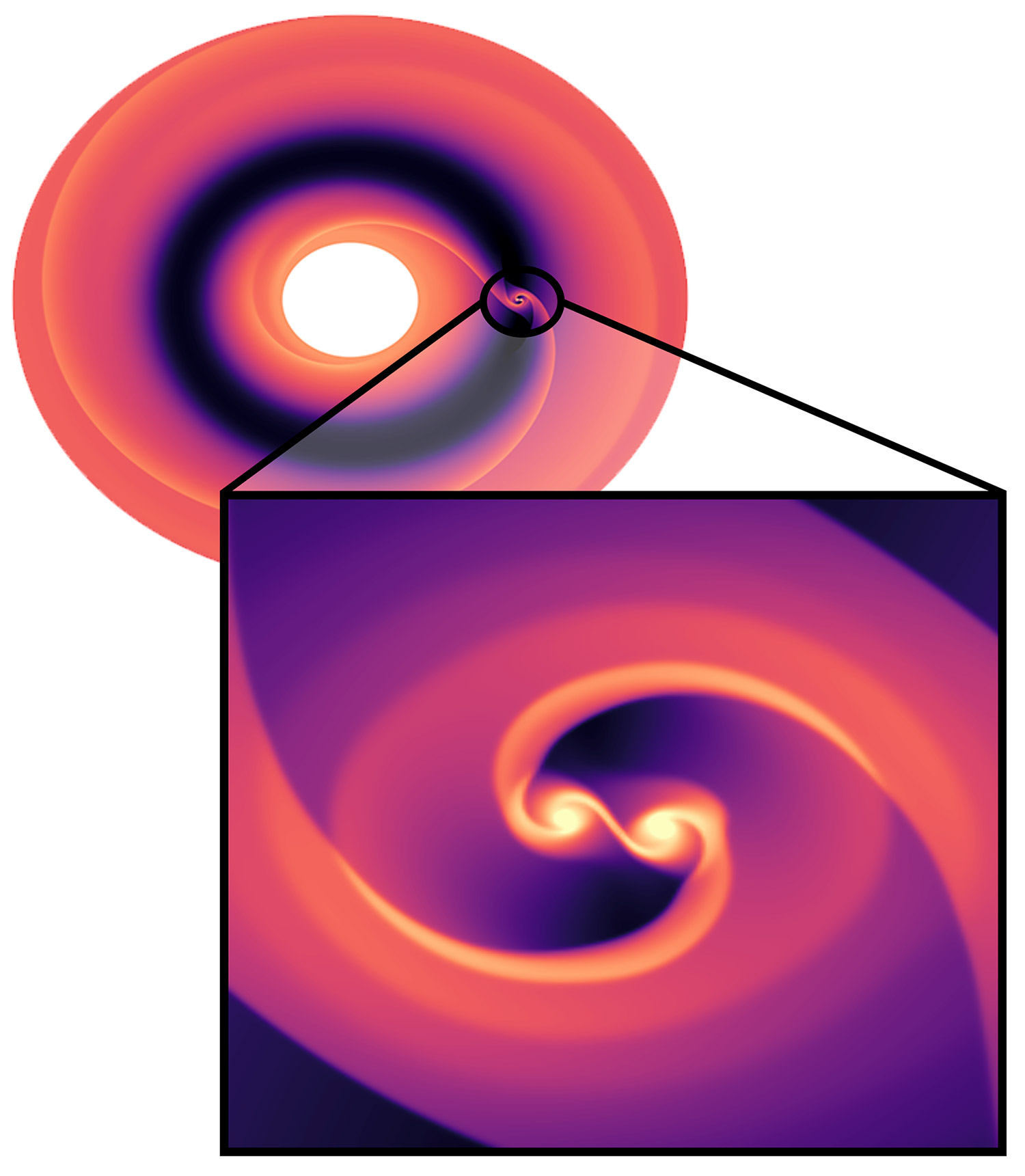
I like this LIGO story a lot, and I’m fortunate to be able to say it’s just the most recent in a long line of similar stories I’ve been involved with over the course of my career. It illustrates something I continuously strive for: recognizing interesting and unexpected possibilities within the emerging scientific theory, simulation, experimentation, and observation. We can now detect energetic phenomena inside the accretion disk of a supermassive black hole in some distant galaxy. And with upcoming multi-messenger observations that allow gravitational waves to be paired with simultaneous telescope observations—visible light or x-rays, for example—the combined signals will reveal details of jets and other high-energy processes at work in the very inner cores of the hidden engines of the universe. For my part, seizing on the possibility that the LIGO binary black-hole merger took place within the magnetized plasma of a much larger black hole’s accretion disk, and working out how it might have played out in detail, is the kind of sleuthing I find deeply satisfying.
Bringing it home
Astrophysics is notorious for being pure research, rather than applied research. Often, applied physics discoveries (such as the properties of nuclear reactions) provide valuable information about what is going on in astrophysical settings, but astrophysics research repays that debt by stretching the parameters to extremes and challenging us to think deeper. And even with the help of applied physics, you still can’t deliberately adjust the settings on a galaxy or a star; you can only try to find one that seems to be already doing what you’re looking for. However, I make a concerted effort to bridge the divide between pure astrophysical research and applied physics and have had some success there.
For example, my collaborators and I conducted MHD studies specifically to help connect astrophysical phenomena with major plasma physics facilities. We demonstrated a number of key parallels between theoretical simulations and experimental outcomes at a plasma-jet experiment at Caltech, and we correlated the astrophysical turbulent magnetic dynamo—the mechanism I mentioned that’s responsible for strengthening magnetic fields in galaxy clusters—with the Omega Laser Facility at the University of Rochester. In each case, we made a class of astrophysical research accessible to controlled laboratory experimentation.
As for repaying the debt to applied physics, magnetohydrodynamically complex plasmas are important from a technological perspective, especially in nuclear-fusion research. We have conducted multiple studies using the MHD simulation capability we developed for astrophysical research to help inform plasma physics generally, including an important subset of energy research known as inertial-confinement fusion. But perhaps my favorite example: We applied sophisticated MHD modeling to the problem of making a strong neutron source (a device called a Dense Plasma Focus)—important in nuclear weapons research—by drawing inspiration from astrophysical jets specifically. If you replace the ordinary hydrogen in the jet gas (hydrogen being the most abundant element in the universe) with the more reactive hydrogen isotopes deuterium and tritium—which produce neutrons when they fuse—and add a strong magnetic field, you get a powerful, magnetically regulated neutron source. Who would have thought we could make the phenomenology of a distant supermassive black hole genuinely useful here on Earth?
So that’s my story: studying the power of magnetism to carry energy far and wide, investigating the ways this power can explain mysterious new discoveries, and grounding it all in rigorous observational, experimental, and even technological science. Honestly, all that is a tall order for a career in astrophysics, and I couldn’t do it on my own. Nor could I do it in a more traditional science job as part of a university faculty. Undeniably, what has made this research path possible is the breadth of talent and resources available to me here at Los Alamos. Outstanding colleagues across divisions—experts in astrophysics, plasma physics, turbulence, high-performance computing, and experiments—and access to world-class supercomputers have made it possible for me to do what I do.
In a way, we are all energized by powerful ideas. We allow them to interact and grow within the turbulent jumble of theories and observations swirling around. Couple these ideas with the talent and inspiration of my mentors, and particularly all the students, postdoctoral researchers, and scientific staff I’ve had the privilege to collaborate with in my Los Alamos career, and we massively extend their reach into the broader scientific enterprise, eroding the mysteries that continue to emerge. LDRD