Sometime in high school, students are taught to balance chemical reactions by selecting the proper coefficients to satisfy conservation of mass. If there were four hydrogen atoms before the reaction, then there must be four after, even though they ended up paired to different elements. However, as I recall, the discussion curiously avoided the topic of how all the atoms traded partners. What are the dynamics of atomic bonding and what dance is performed in the process? I might have imagined one atom squeezing instantaneously between an existing pair, but the richness of nature is rarely captured by simple intuition. So how might we directly study or observe the dynamics of chemistry? What are the transient intermediate states of bond formation? Until recently, scientists have not been able to address these types of questions apart from computational models and limited experimental studies.
Also in high school, I became fascinated by the thought of one day working at a national laboratory. I grew up in rural Maryland, seemingly far removed from the facilities and opportunities of a national lab. A series of amazing events (credited to dedicated educators) allowed me to participate in several summer-long internships at Oak Ridge National Laboratory in Tennessee and what is now the Thomas Jefferson National Accelerator Facility in Virginia. I got to participate in ongoing experiments led by world-renowned scientists and even co-presented some of the results at scientific conferences. I remember being delighted to learn that people were paid to be inquisitive and curious!
As I pursued my own scientific career at a national lab, I came to appreciate that a great deal of present and future scientific discovery relies critically on what was not covered in chemistry class: the dynamics of the atomic scale. Most of the big challenges for humans—from understanding and treating disease to advancing and stewarding modern weapons—hinge upon dynamically observing and sometimes controlling matter on the atomic scale. Indeed, over the past decade at Los Alamos, seeing what atoms are doing has emerged as a key focus of my career.
Illuminating the frontier
Across the arc of human discovery, from our ancestors seeking to understand their surroundings to contemporary scientists hoping to observe the secret lives of atoms, a common challenge persists: obtaining a suitable light source to probe the unknown. For a long time, our species wrestled with the problem of light-generation technology (e.g., fashioning a torch to see inside a cave or reflecting sunlight to illuminate a microscope stage). Now, that struggle has evolved to the point of producing high-energy x-rays to observe the next frontier in human understanding: the dynamic world of molecules and atoms.
Why do we need such a specific light source? There are many reasons. We need x-rays because their wavelength (related to their energy) is suitable to the atomic scale in a way that visible light is not. Atoms vary in size but are generally nanometer-scale (billionths of a meter), and one must go smaller still to discern the way their electrons are arranged. Lower-energy, or “soft,” x-rays have small enough wavelengths to access the former, and “hard” x-rays can accomplish the latter. The wavelengths of visible light, on the other hand, are hundreds or thousands of times too big. Trying to examine an individual atom with visible light is like trying to examine an individual grain of sand by poking at the beach with a baseball bat. The size of the probe does not nearly match the size of the target under study.
Light sources are a modern crossroads, routinely bringing together theorists, experimentalists, and computational scientists.
X-ray imaging is already a powerful technique because x-rays can penetrate solid matter and have a wavelength short enough to resolve interatomic distances via the wave-interference phenomenon of diffraction. Hard x-rays have the added benefits of accessing electrons close to atomic nuclei, to get a more complete picture of the atom (rather than just its outermost electrons), and their limited interaction with matter prevents radiation damage to the target being observed. But hard x-rays are just energetic photons, so what other characteristics of the x-ray light are needed to observe atomic dynamics?
We need x-rays that are both monochromatic (all one wavelength) and coherent (synchronized, cresting and falling together); these are the properties of a laser. A red laser, for instance, isn’t just red; it’s a very, very narrow shade of red, with all of its light waves in phase. Similarly, the x-ray laser we need would span a very narrow slice of the x-ray band. This is necessary to produce a sharp image we can understand. Using incoherent x-rays of uncertain wavelength would be analogous to trying to make a measurement with a ruler that has uncertain units of length.
In addition, our laser must be extremely bright. About 99.9 percent of x-ray photons will pass through the sample without interaction, which means the remaining 0.1 percent has to do the whole imaging job. And only a very bright laser would be capable of probing many important sample materials at the length scales of interest. Traditionally, x-ray diffraction only works on a large, macroscopic crystalline sample, but a bright enough x-ray laser could function effectively on smaller targets—even individual molecules that resist crystallization, such as various enzymes.
Finally, to observe the time dynamics of atomic bonding, the laser pulses will have to arrive in very brief, rapid bursts—on the order of a femtosecond, a millionth of a billionth of a second. So the challenge before us is to make a high-energy, coherent, monochromatic x-ray light source to illuminate the time-dynamic behavior of atoms. Light sources have undergone tremendous advances, yielding rich rewards and discoveries over the decades. But to make the leap to next-generation sources of hard x-rays, we need just that, a leap.
Critical cathode
There is a way to produce the copious hard x-rays we need, and fortunately, it is suitable for making those x-rays shine as a laser. We start with a beam of electrons traveling at nearly the speed of light and use a sequence of alternating magnets called an undulator to make the electrons wiggle from side to side as they travel. When charged particles accelerate (e.g., as part of the wiggling motion), they emit electromagnetic radiation, called synchrotron radiation, in order to conserve energy and momentum. By tightly controlling the energy of the electrons and the strength and spacing of the magnets, we can select the desired x-ray wavelength for the emitted radiation.
Because an electron in the beam is moving at nearly the speed of light, there is a condition in which its back-and-forth wiggle motion allows it to slip behind its emitted synchrotron radiation by exactly one wavelength with every wiggle period. This means that photons generated by one electron near one magnet will add coherently to other photons generated by the same electron at every other magnet. This helps increase brightness, but it would be much preferred if emission from every electron would remain coherent with that of all the other electrons, as in a laser.
The only way to make all the electrons in a beam radiate in phase like a laser is to have them undergo identical wiggling motion. This, in turn, requires that they be packed into a tightly defined bunch, much like people packed in a single car of a rollercoaster—all the people undergo the same acceleration at the same time. This concept is the basis of the “free-electron laser”: the emitted synchrotron radiation interacts with the electrons, causing them to bunch up and assume nearly identical positions. The radiation emitted by the bunched-up electrons causes them to bunch up even more, with bunch spacing equal to radiation wavelength, which results in the electrons emitting x-rays that are coherent, and the feedback process producing a gain in intensity. This is known as an x-ray free-electron laser (XFEL)—a device capable of a million times more energy per pulse and 10,000 times shorter pulse durations than previous‑generation, non-laser synchrotron light sources.
While it is relatively straightforward to design the array of wiggler magnets, launching and preserving (during acceleration) a sufficiently uniform beam of electrons—one that ultimately yields coherent, monochromatic x-rays—is much more difficult. A beam of electrons can be produced from a specialized-material component known as a cathode, which can be made to emit electrons by applying energy in the form of incident light. We bombard our cathode with photons from a precisely timed drive laser to obtain electrons, which we then accelerate using radio-frequency electromagnetic fields. Yet if the electrons are to be sufficiently identical in energy and direction for an XFEL, the cathode material must be essentially perfect. In fact, our collaborative team relies upon the materials science techniques of x-ray diffraction, reflection, and fluorescence to tune and perfect the photocathode (light‑driven cathode) growth process in real time.
The point is, while an XFEL has many sophisticated components, the entire system performance must be designed to accommodate the constraints of cathode performance. And while research directions for XFELs are diverse, all share the same present and future need for high-quality electron sources that scale to evolving demands. This is both a challenge and an opportunity, since improving cathode technology can translate to better light-source performance for other applications as well, such as ultra-fast electron diffraction and microscopy.
Unconventional materials
My research team focuses on solving the problems involved in getting a cathode to emit a very bright electron beam by hitting it with incident light. While brightness is an intuitive term, it is also a metric defined by the number of electrons emitted from a given area with a given energy and a narrowly defined direction. Essentially all applications would benefit from the brightest possible beam, but it turns out that the physics of photoemission in conventional materials prevents the individual optimization of efficiency, time response, beam quality, and lifetime. In other words, improving one of these properties to the degree necessary tends to compromise one or more of the others. This limits the methods to optimize cathode performance for specific applications—such as Los Alamos’s upcoming Matter-Radiation Interactions in Extremes (MaRIE) materials-research facility and other advanced XFELs—because improvements in efficiency tend to increase the divergence and energy spread of the beam at the cathode (which is bad because the electrons become less identical). The strategy we have adopted is to apply a materials-science focus to optimizing photoemission performance. In particular, we influence the underlying mechanisms of photoemission in a predictable way with the goal of simultaneously optimizing each performance metric or at least achieving new tradeoffs that are beneficial.
While an XFEL has many sophisticated components, system performance is limited by the cathode.
To do this, we need to control the most fundamental properties of the photocathode material—its electronic structure. That electronic structure governs all three steps in the process of photoemission: absorption of the incident photon by an electron in the material, movement of that electron to the surface of the cathode, and extraction of the electron into free space. In conventional approaches, these processes cannot be significantly altered or improved. This is what led us to focus on novel techniques and materials.
There are various ways to manipulate a material’s electronic structure, but they are largely in their infancy, with most of the innovations coming from Los Alamos. For example, quantum dots, nanometer-sized semiconductor crystals, exhibit electronic absorption and transport that is very different from macroscopic materials. They can be engineered to favor a particular wavelength for absorption and simultaneously situate the absorbing electron near the surface to maximize extraction efficiency. The small size of the nanocrystal also naturally confines the range of energy of emitted electrons; these are effects we have recently demonstrated and published.
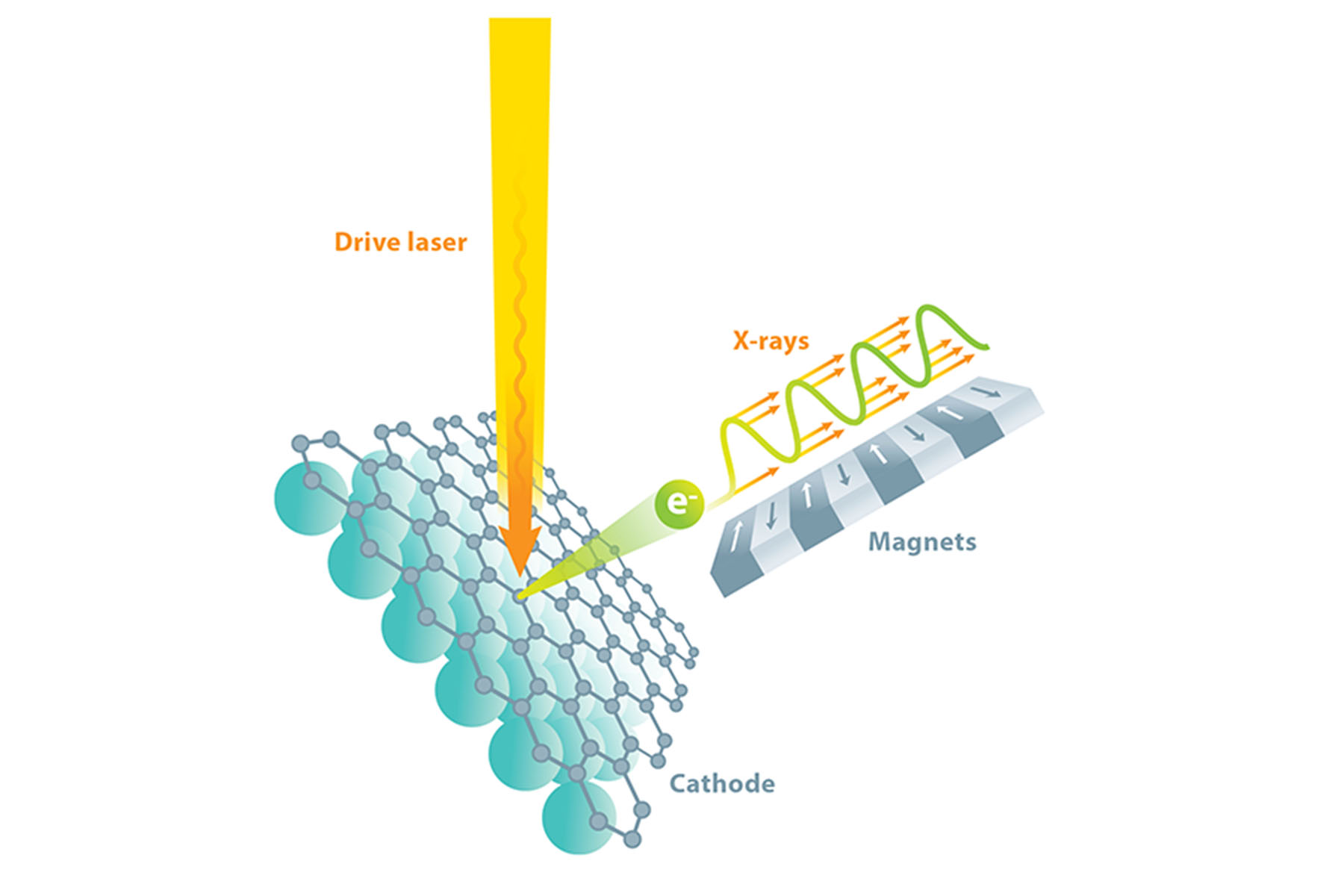
We are also tailoring existing materials using new growth methods that yield atomic smoothness over a large crystal grain size. In addition, we have recently demonstrated how graphene and other single-molecule-thick surface coatings can be used to improve the lifetime of a cathode in a practical operating environment without significantly altering its photoemission response.
We are exploring many more ideas too, with an emphasis on closely integrating exciting developments in materials science with those of the beam-physics community. The ability to tune a material for specific photoemission applications opens up new areas of research that are just beginning to be explored. For advanced accelerator applications, these techniques allow us to envision a path to designing custom photocathodes that match the requirements of a new accelerator or enable a significant upgrade of one that already exists. Applications outside of large accelerator sources will likely benefit from these advances as well, and our team holds four patents in these areas of innovation.
What an XFEL can do
The fundamental questions targeted by investigations using XFELs involve the motion of charge and its interaction with atomic nuclei: How do atoms and molecules move when chemical bonds are formed and broken? What are the pathways of radiation-induced motion, and what constitutes damage to a molecule or material? Atomic nuclei in molecules move distances comparable to the XFEL wavelength (less than a nanometer) in femtoseconds, and valence electrons move about within an interatomic bond in less than a femtosecond, so the femtosecond-pulsed hard x-ray radiation from an XFEL directly matches these dimensions of space and time. The bursts of XFEL pulses allow stroboscopic study of this motion. The envisioned result is effectively a molecular movie showing atom-to-atom detail of time evolution of electronic charge in bonds, which will reveal phenomena not otherwise discernable or understood.
One set of potential applications seeks to pinpoint the origin of disease and cellular malfunction by deciphering the molecular composition of cells and membranes as well as their dynamical motion. The human genome project has identified approximately 25,000 genes in human DNA that regulate all aspects of life, such as respiration, digestion, immunity, and reproduction. Studying these genetic structures and their role in protein synthesis with an XFEL may help identify the origin of specific malfunctions that give rise to disease. Such knowledge could lead to new techniques in disease prevention and treatment. And just as with DNA, knowing and observing the structure of other biomolecules is an integral part of discovering their function as well.
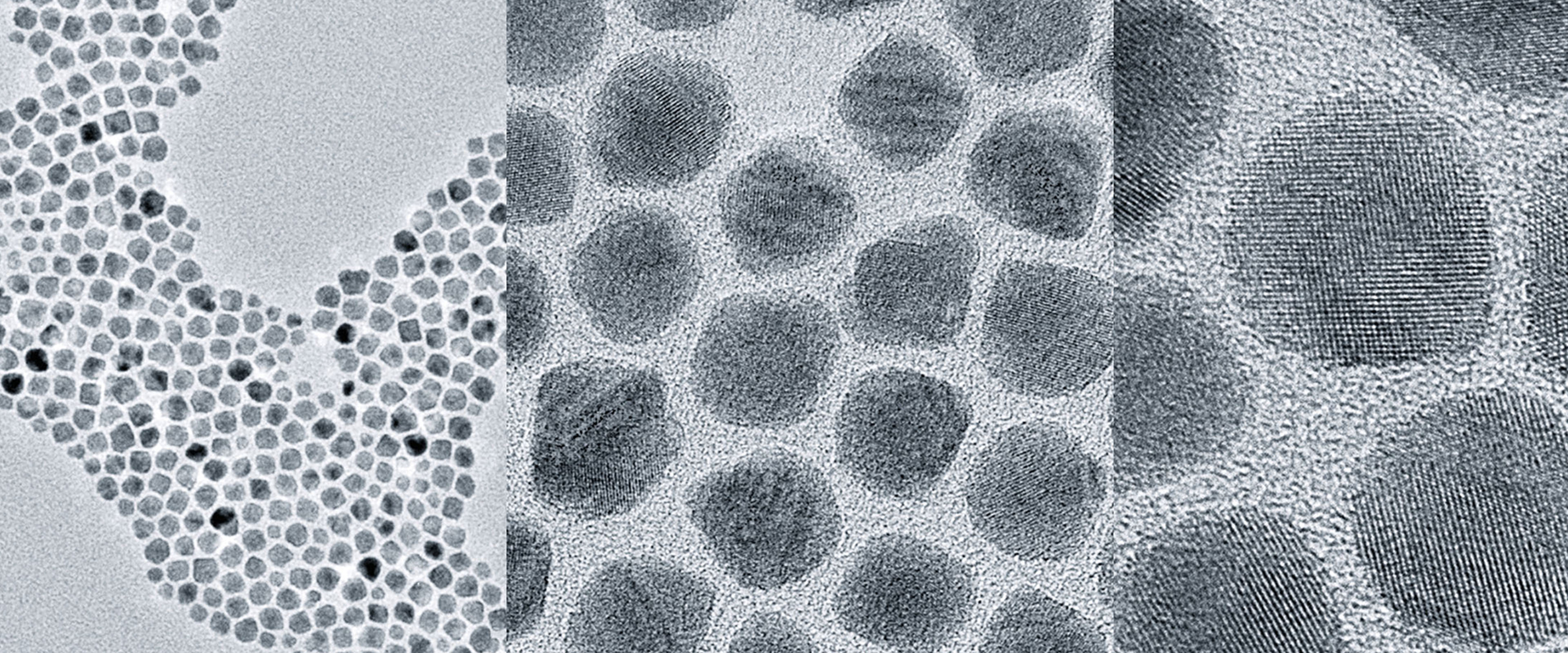
shown here in successive magnification, can be designed to absorb a specific frequency of laser light and transport electrons to the material’s surface for emission as a beam.
Another major class of applications concerns catalysis, the study of directed chemistry—enabling, accelerating, controlling, or otherwise optimizing chemical reactions using agents known as catalysts. Observing how these agents interact at the molecular level in many industrial chemical processes could allow drastic reduction of toxic waste and byproducts and offer more efficient use of resources. One example is the study of the molecular composition of soot to improve combustion efficiency; another is the development of chemical processes that mimic the energy-harvesting process of photosynthesis.
Applications span many disciplines, including biology, medicine, pharmacology, chemistry, materials science, energy research, environmental research, electronics, photonics, nanotechnology, astrophysics, and fundamental physics.
Other applications include understanding the unique properties of natural systems, such as the microstructure of body armor in nature, e.g., an abalone shell. The iridescent abalone shell consists of a stacked structure of proteins and calcium carbonate, which makes it 3000 times more fracture resistant than crystals of the same material. Observing material boundaries of this and many other natural materials at the mesoscale (the range of dimensions between billionths and millionths of a meter) and under dynamic conditions would allow researchers to understand and more closely mimic these properties in synthesized materials.
In materials science, the dynamics of atomic and molecular bonding dictates the landscape of the mesoscale, where the collective behavior of many atoms sets the macroscopic properties needed for real-world applications. It is reasonable to anticipate that XFEL-based materials research will lead to improved, smaller, and more efficient electronic components, solar cells, fuel cells, and even self‑healing materials.
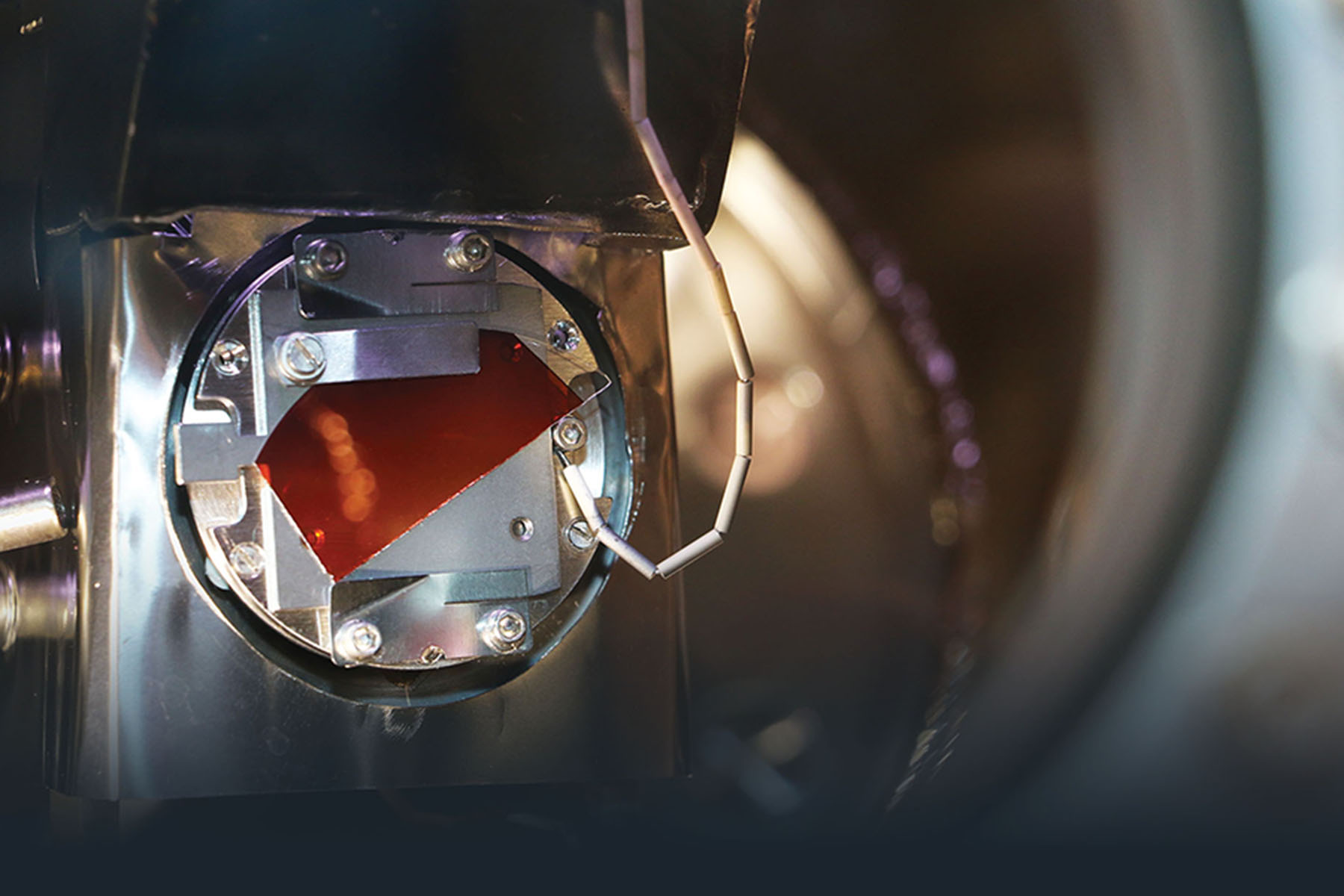
Existing XFELs allow for the study of atoms, molecules, large non-crystalline particles (bio-molecules), liquids, and soft and condensed matter. But to make such measurements at the mesoscale, at which interactions between grains in the material dominate the material’s performance, requires focusing these intense x-ray laser beams to small spots. Existing XFELs generate relatively long x-ray wavelengths that are totally absorbed in a thick sample of all but the lightest elements, with the sample vaporized by a single pulse at the intensities required for mesoscale measurements.
The key features of the XFEL that we are supporting are two-fold: producing shorter-wavelength x-rays and generating a string of pulses in a very short period of time. By generating x-rays that have five to ten times shorter wavelengths, the x-ray absorption and the resulting heating is one to two orders of magnitude less, and the properties of materials made from both light and heavy elements can be measured without destroying the sample. This reduced heating also enables taking “movies” with a string of closely spaced pulses on thick samples undergoing fast changes, such as those that occur during welding, additive manufacturing (3D printing), and explosively driven shocks. This new XFEL capability will lead to improvements in material production, optimizing material properties to best meet various needs. The benefits to the Lab’s nuclear weapons stockpile-stewardship mission alone would be substantial both in performance and cost savings.
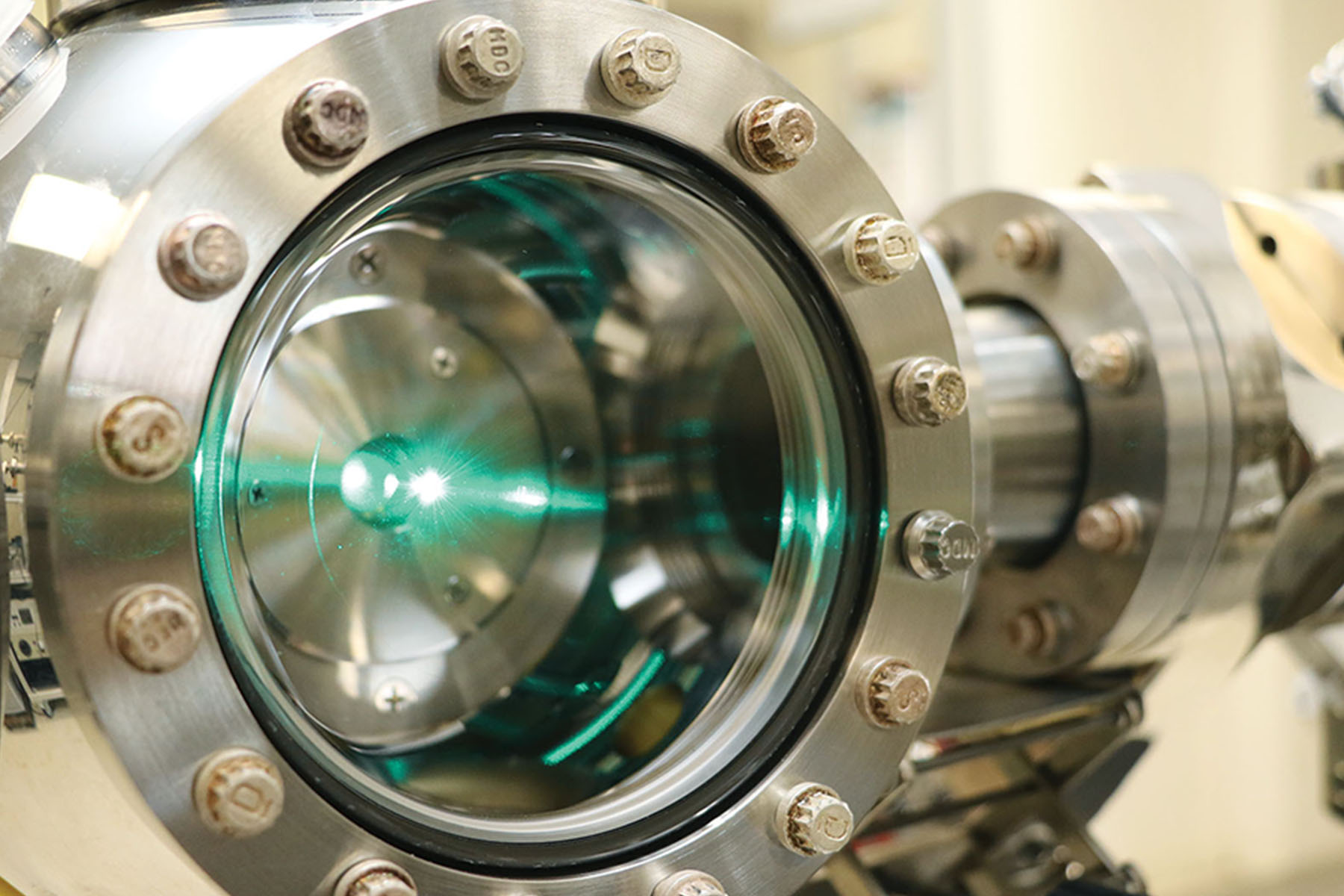
To move forward, we’re benefitting from a new partnership between materials science and accelerator physics. Light sources are a modern crossroads for sharing significant ideas and findings, routinely bringing together theorists, experimentalists, and computational scientists from multiple disciplines around a single study. Indeed, I am convinced that this is the way the greatest scientific advances will continue to happen. The future ahead of us is going to come about by interdisciplinary science.